
An official website of the United States government
The .gov means it’s official. Federal government websites often end in .gov or .mil. Before sharing sensitive information, make sure you’re on a federal government site.
The site is secure. The https:// ensures that you are connecting to the official website and that any information you provide is encrypted and transmitted securely.
- Publications
- Account settings
Preview improvements coming to the PMC website in October 2024. Learn More or Try it out now .
- Advanced Search
- Journal List
- Springer Nature - PMC COVID-19 Collection


COVID-19: impact on Public Health and hypothesis-driven investigations on genetic susceptibility and severity
Susana david.
1 Departamento de Genética Humana, Instituto Nacional de Saúde Doutor Ricardo Jorge (INSA,IP), Lisboa, Portugal
2 Instituto de Investigação do Medicamento (iMed.ULisboa), Faculdade de Farmácia, Universidade de Lisboa, Lisboa, Portugal
Guillermo Dorado
3 Atlántida Centro de Investigación y Desarrollo de Estudios Profesionales (CIDEP), Granada, Spain
Elsa L. Duarte
4 MED-Instituto Mediterrâneo para a Agricultura, Ambiente e Desenvolvimento, Escola de Ciências e Tecnologia, Universidade de Évora, Évora, Portugal
Stephanie David-Bosne
5 Université Claude Bernard Lyon 1, Lyon, France
João Trigueiro-Louro
6 Departamento de Doenças Infeciosas, INSA, IP, Lisboa, Portugal
7 Host-Pathogen Interaction Unit, Instituto de Investigação do Medicamento (iMed.ULisboa), Faculdade de Farmácia, Universidade de Lisboa, Lisboa, Portugal
8 Hospital Egas Moniz, Centro Hospitalar Lisboa Ocidental, Lisboa, Portugal
Helena Rebelo-de-Andrade
COVID-19 is a new complex multisystem disease caused by the novel coronavirus SARS-CoV-2. In slightly over 2 years, it infected nearly 500 million and killed 6 million human beings worldwide, causing an unprecedented coronavirus pandemic. Currently, the international scientific community is engaged in elucidating the molecular mechanisms of the pathophysiology of SARS-CoV-2 infection as a basis of scientific developments for the future control of COVID-19. Global exome and genome analysis efforts work to define the human genetics of protective immunity to SARS-CoV-2 infection. Here, we review the current knowledge regarding the SARS-CoV-2 infection, the implications of COVID-19 to Public Health and discuss genotype to phenotype association approaches that could be exploited through the selection of candidate genes to identify the genetic determinants of severe COVID-19.
Introduction
COVID-19 is a new, complex, multisystem disease caused by the novel coronavirus SARS-CoV-2. In slightly over 2 years, it infected nearly 500 million and killed 6 million human beings worldwide, causing an unprecedented coronavirus pandemic. Several vaccines, developed in record time, were approved for public use (Forni and Mantovani 2021 ). However, their real-world effectiveness in controlling the COVID-19 pandemic is still under debate. Consequently, other scientific approaches to control the disease are also needed. The wide spectrum of interindividual variability in the clinical manifestation of COVID-19, ranging from asymptomatic infection to severe disease and death, has gone unexplained by mutations in SARS-CoV-2. Therefore, notwithstanding underlying comorbidities and risk factors, host genetics appears to be a possible source and major contributor of these observed differences in the host–pathogen relationship. The identification of genetic determinants of COVID-19 susceptibility and severity could represent a valuable tool in the control of COVID-19.
In this review, we begin by summarizing recent investigations regarding the epidemiology and Public Health of COVID-19. We then describe the biology and pathogenesis of the SARS-CoV-2 and the immune response to this virus in humans. Finally, we review sources available for candidate gene selection and hypothesis driven investigations on the genetic susceptibility and severity of COVID-19.
An overview of the Public Health impact of SARS-COV-2
Coronaviruses (CoVs) constitute a worldwide threat to Public Health. CoVs may cause respiratory, enteric, hematological, and neurological disorders, to name a few. They are able to infect many animal species, also ranging from asymptomatic to severe clinical forms. There is great concern to the fact that the newly emerging CoVs have demonstrated the capacity to jump across species barriers with animal‐to‐human transmission, also acquiring the ability of efficient human‐to‐human transmission (Li 2016 ; Phan et al. 2018 ; Chen et al. 2020 ).
The previous CoV epidemic outbreaks include the 2002/2003 outbreak of Severe Acute Respiratory Syndrome (SARS)-CoV (also reported as SARS-CoV-1). Like SARS-CoV-2, it also originated in China (Guangdong). (SARS)-CoV spread to nearly 30 countries and infected more than 8000 people, causing about 1000 deaths, with an 11% fatality rate (World Health Organization, WHO 2006 ). The 2012 outbreak of Middle East Respiratory Syndrome (MERS)‐CoV, from Saudi Arabia, also originated cases in nearly 30 other countries and was responsible for over 2000 cases and nearly 1000 deaths, with an approximate 35% fatality rate (Donnelly et al. 2019 ). The (SARS)-CoV-2 has surpassed by several orders of magnitude these earlier CoV outbreaks in the number of cases and deaths. Compared to the previous outbreaks (more confined in time and space), the SARS-CoV-2 outbreak, that started in 2019 in Wuhan, China, has plundered global health as well as the economic and social sectors, in an unprecedented CoV pandemic.
The spread of the SARS-CoV-2 to all continents occurred at a fast pace. On December 31, 2019, the health authorities of Wuhan reported 27 people diagnosed with a pneumonia of unknown origin. In the following days, reports of cases appeared in Thailand, Japan, and the USA, all of them from people who had recently traveled to Wuhan or had been in contact with an infected person (supporting the ability of sustained human-to-human transmission) (Park 2020 ). On March 11, 2020, in view of its rapid expansion and high fatality rate, the World Health Organization (WHO) announced the SARS-CoV-2 infection as a pandemic (WHO Mar 11, 2020 ). In less than 1 year, the virus had already infected over 50 million individuals and resulted in the loss of more than one million lives (Dong et al. 2020 ; WHO November 17, 2020 ).
It is worth highlighting that the SARS-CoV-2 viral genome was isolated and sequenced at an unequalled pace and that the results became readily available within 3 days after the first coronavirus cases were reported (Genebank accession {"type":"entrez-nucleotide","attrs":{"text":"MN908947","term_id":"1798172431","term_text":"MN908947"}} MN908947 ) (Wu et al. 2020a ). Three months later, GeneBank had more than five hundred genomic sequences of the virus from samples collected in different parts of the world.
COVID-19 has negatively affected several aspects of Public Health. The diversion of the healthcare workforce to the mitigation of SARS-CoV-2, indirectly led to the neglect of measures regarding both communicable and non-communicable diseases. This has been the case for humanity’s main infectious disease threats: tuberculosis (TB); human immunodeficiency virus (HIV) and acquired immunodeficiency syndrome (AIDS); and malaria. Ending these epidemics by 2030 is among the health targets of the 2030 Sustainable Development Goals. However, in the wake of the SARS-CoV-2 pandemic, the milestones are at risk of a substantial regression. Studies modeling the current situation, suggest a potential setback of five to eight years in the fight against the incidence and deaths from TB, 20 years in the fight against mortality due to malaria and about 12 years in AIDS-related deaths (Cilloni et al. 2020 ; Jewell et al. 2020 ; Stop TB Partnership 2020 ; WHO 2020 ). These top killer infectious diseases concentrate in current high burden countries mainly in Asia and sub-Saharan Africa, whereas the SARS-CoV-2 has taken its toll on high- and low-income countries alike. Perhaps surprisingly, some high-income economies have been among the hardest hit.
For the SARS-CoV-2, the secondary attack rate was estimated to exceed 10% (Burke 2020 ; Ghinai et al. 2020 ). A systematic review and meta-analysis reported a household secondary attack rate for the SARS-CoV-2 of 18%, higher than the upper range of estimates for the 2009 H1N1 pandemic influenza (5–15%) and also higher than that observed for both SARS (5–10%) and MERS (4–5%) (Koh et al. 2020 ). Moreover, there is the possibility of superspreading events (SSEs), with reports of over 50% and 80% attack rates among confirmed and all cases, respectively (Hamner 2020 ). Another key aspect is that all the observed forms of COVID-19’s clinical presentation, from asymptomatic to symptomatic; appear to be potentially infectious (Bai et al. 2020 ; Chau et al. 2020 ; Hoehl et al. 2020 ; Yu et al. 2020 ).
The relationship between viral load and infectivity or disease severity remains unclear (Walsh et al. 2020 ). Increased viral load of index cases appears as a leading driver of SARS-CoV-2 transmission (Marks et al. 2021 ). However, whereas in some studies high viral load appears to be associated with immune factors, disease severity and mortality (Fajnzylber et al. 2020 ; Pujadas et al. 2020 ; Shenoy et al. 2021 ), others have reported conflicting results, namely in children and asymptomatic patients (Hasanoglu et al. 2021 ; Aykac et al. 2021 ). Strain-specific differences have also been reported (Luo et al. 2021 ; He et al. 2021 ). Currently, no standard measure of viral load in clinical samples is available (Dahdouh et al. 2021 ); therefore, the relationship between viral load and infectivity or disease severity needs to be further investigated. Moreover, evidence from the literature does not directly suggest that severity would be only mediated by viral load but also by age and the impact that age has on the immune system (Chou et al. 2022 ).
“Host-jumps” in CoV appear to result in short-term spillover infections, with little evidence for sustained onward transmission in the new host species (Leopardi et al. 2018 ). However, this was not the case in the recent SARS-CoV-2 outbreak in minks, where sustained then back to human infection was observed (ECDC 2020 ; WHO January 20, 2021 ). In the earlier CoV outbreaks of SARS and MERS, the early SSEs gave way to sustained transmission in the later stages of the epidemics. With the possibility of SSEs and both symptomatic and asymptomatic transmission of the virus, the 1-year fatality rate from the SARS-CoV-2 pandemic has surpassed the levels observed for malaria, HIV/AIDS, influenza virus and TB, the deadliest pre-COVID-19 infectious disease of humanity in modern times (Table (Table1). 1 ). It has been suggested that the SARS-CoV-2 may represent the fifth CoV to be introduced and become permanently established in the human population, along with the prior four seasonal CoVs that currently circulate in humans (Escobedo et al. 2022 ; Li et al. 2021 ). Ultimately, this will be mainly determined by the forthcoming interplay between the host (e.g., immunity and susceptibility to the virus), the environment and the viral pathogen (e.g., potential emergence of newly SARS-CoV-2 variants) (Escobedo et al. 2022 ; Li et al. 2021 ).
Comparative burden on human life of five major infectious diseases (1 year estimates)
TB tuberculosis, M. tuberculosis Mycobacterium tuberculosis , HIV human immunodeficiency virus, AIDS acquired immunodeficiency syndrome, SARS severe acute respiratory syndrome, CoV coronaviruses, N number of, sp. = Species; fatality rate = N. deaths/ N. cases (%), EMR mean influenza-associated respiratory excess mortality rate
1 In 2018, the 30 high TB burden countries accounted for 87% of new TB cases. Eight countries account for two thirds of the total number of cases, led by India, followed by, China, Indonesia, the Philippines, Pakistan, Nigeria, Bangladesh, and South Africa. Number of cases corresponds to the 2018 incidence rate. Number of deaths reported for 2018. https://www.who.int/news-room/fact-sheets/detail/tuberculosis (accessed on June 11, 2020)
2 In 2017 approximately 93% of all deaths were in the WHO African Region and almost 80% of all deaths in 2017 occurred in 17 countries in the WHO African Region and India. Number of cases corresponds to the 2018 incidence rate. Number of deaths reported for 2018. https://www.who.int/gho/malaria/en/ (accessed on June 11, 2020)
3 Sub-Saharan Africa remains the most heavily affected region of the world, accounting for approximately two thirds of all incident and prevalent HIV infections and three quarters of all AIDS deaths. Number of cases corresponds to people living with HIV in 2018. Number of deaths reported for 2018. https://www.who.int/gho/hiv/en/ (accessed on June 11, 2020)
4 Estimate relative to the first year of the COVID-19 pandemic. Almost a year after the initial report by the health authorities in Wuhan, China, on December 31, 2019, the SARS-CoV-2 pandemic reached 190 countries, surpassing the 2018 mortality from malaria, HIV/AIDS and viral influenza, and rapidly approaching that of the most deadly infectious disease, tuberculosis. https://www.arcgis.com/apps/opsdashboard/index.html#/bda7594740fd40299423467b48e9ecf6 (accessed on November 17, 2020)
5 In a sample representing 57% of the global population, EMR ranged from 0.1 to 6.4 per 100,000 individuals for people younger than 65 years, 2.9 to 44.0 per 100,000 individuals for people aged between 65 and 74 years, and 17.9 to 223.5 per 100,000 for people older than 75 years (Iuliano et al. 2018 ). https://www.who.int/en/news-room/fact-sheets/detail/influenza-(seasonal ) (accessed on June 11, 2020)
Human-tropic COVS
The CoVs belong to the Coronaviridae family and the Orthocoronavirinae subfamily. They are the largest of the RNA viruses, carrying a positive-sense RNA strand of approximately 26–32 kilobases. The CoVs are spherical or pleomorphic in shape and approximately 125 nm in diameter with surface club-shaped projections, similar to what would resemble a crown, from hence the name (from the Latin “corona,” meaning “crown”) (Chen et al. 2020 ). These enveloped RNA viruses replicate in the host cell cytoplasm and antagonize the interferon and other host proteins of the innate immune response.
CoVs consists of four serologically and genetically differentiated genera, the Alphacoronaviruses ( Alpha-CoV ), Betacoronaviruses ( Beta-CoV ), Gammacoronaviruses ( Gamma-CoV ), and Deltacoronaviruses ( Delta-CoV ). CoVs infect over 12 mammalian and avian species and demonstrate the capacity to cross the species barrier. They generally exhibit host genus-specificity, characterized by a narrow host range, infecting one or just a few species dictated by the viral interaction with the corresponding host cell receptor (Leopardi et al. 2018 ). There are seven known human CoVs. These belong to the Alpha-CoV and Beta-CoV genus. The CoV-NL63 and CoV-229E are representative human Alpha-CoVs . Human Beta-CoVs include SARS-CoV-2, SARS‐CoV, MERS‐CoV, CoV-HKU1, and CoV-OC43. Three of these species are particularly pathogenic to humans: the SARS-CoV, its closely resembling SARS‐CoV-2, and the MERS‐CoV. SARS-CoV and MERS‐CoV have originated from bats with intermediate hosts identified as civet cat and dromedary camels; respectively (Walls et al. 2020 ). The origin of SARS-CoV-2 is still controversial. The remaining endemic species HCoV-229E, HCoVNL63, HCoV-OC43 and HCoV-HKU1 are the known cause of common colds.
CoVs are composed of four basic structural proteins in their lipid bilayer envelope, the spike (S), envelope (E), membrane (M), and nucleocapsid (N), encoded by one-third of the CoV genomic RNA. The role of the spike glycoprotein (S) is particularly relevant as the determinant of species specificity in CoV infections (Kuo et al. 2000 ), and has been explored as the major anti-CoV target (Trigueiro-Louro et al. 2020 ).
SARS-COV-2 infection
Although SARS-CoV-2 is a new challenge, similarities with other CoVs set the groundwork for dissecting the immunological pathways that come to play in COVID-19 (de Wilde et al. 2018 ; Li et al. 2020a ; Ortiz-Prado et al. 2020 ; Vabret et al. 2020 ). SARS-CoV-2 pathophysiology is being extensively investigated in the biological pathways governing the early stages and characterizing the immune responses to the infection. These pathophysiological mechanisms underpin the host genetic determinants of the disease.
The early stages of SARS-CoV-2 infection
In a comparative study carried out by Hui and collaborators, SARS-related CoVs showed a natural tropism for the human respiratory tract, although also targeting extrapulmonary cells, such as the vascular endothelial cells (Hui et al. 2020 ). The early stages of the infection process for SARS-CoV-2 are similar to those described for SARS-CoV, thus: 1) the viral S1 N-terminal domain of the S protein attaches to the angiotensin-converting enzyme 2 (ACE2), the targeted host cell surface receptor of the virus (Hoffmann et al. 2020 ; Walls et al. 2020 ; Zhou et al. 2020 ); 2) redundant proteolytic pathways ensure S protein activation required for cell entry. The S protein acts as the leading mediator of viral entry, binding the virus to the target cell receptor and allowing fusion of the viral envelope with the virus-target cell membrane (Belouzard et al. 2012 ; Heald-Sargent and Gallagher 2012 ; Trigueiro-Louro et al. 2020 ). CoV S proteins are class I membrane fusion proteins, synthesized as inactive precursors, needing proteolytic activation (Kielian and Rey 2006 ). The ectodomain of the S protein consists of two subunits, an S1 N-terminal domain responsible for receptor binding and an S2 C-terminal domain responsible for membrane fusion. The two domains are joined by the protease sensitive S1-S2 junction. Two proteolytic pathways assist the virus in entering the cell: the endosomal pathway, characterized by the proteolytic activity of the endosomal cysteine proteases cathepsins (CTS), such as cathepsin L (CTSL) (Simmons et al. 2005 ), and/or the cell surface non-endosomal pathway, activating the transmembrane protease serine 2 (TMPRSS2) and the human airway trypsin-like (HAT) proteases (Chan et al. 2015 ). Moreover, as with the MERS-CoV, the proprotein convertase furin appears to preactivate S protein cleavage (Millet and Whittaker 2014 ), whereas in SARS-CoV the furin-like cleavage site is absent (Coutard et al. 2020 ). These factors typically co-express in the target cells contributing to the tissue tropism of the virus.
Increased transmissibility of SARS-CoV-2
Despite the similarities between the infection process of SARS-CoV-2 and SARS-CoV, major differences between the two viruses have been identified. Several factors support the increased transmissibility of SARS-CoV-2 in comparison to SARS-CoV. A greater affinity for the ACE2 receptor binding domain (RBD) of SARS-CoV-2 compared to that of SARS-CoV may account for a more efficient cell entry (Shang et al. 2020a ). In SARS-CoV-2 the sequence of the S1-S2 junction shows greater affinity for the host proteases that activate the S protein function, than in SARS-CoV (Meng et al. 2020 ). Moreover, the activation of the SARS-CoV-2 S protein by furin, abundantly expressed in the lungs, reduces the dependence of the virus on the target cell surface protease, TMPRSS2, and the lysosomal proteases, CTSs (Shang et al. 2020b ). Interestingly, the SARS-CoV-2 genotypes more ancestrally related to CoVs in animals are being replaced by still emerging genotypes presenting higher transmissibility in humans (Tang et al. 2020 ; Karim and Karim 2021 ).
Challenges in the face of the new SARS-CoV-2 variants
Key mutations within the Spike protein affecting the biological functions of SARS-CoV-2 led to the emergence of the viral genetic variants that have been circulating worldwide and contribute to different phenotypes of COVID-19 upon infection by these variants (Osuchowski et al. 2021 ; Zhou and Wang 2021 ). The World Health Organization (WHO) along with the Centers for Disease Control and Prevention (CDC) currently define four classes of SARS-CoV-2 variants, namely: Variants under Monitoring (VUM), Variants of Interest (VOI), Variants of Concern (VOC) and Variants of High Consequence (VOHC) (CDC 2021 ; WHO 2022 ). Currently, there are no variants categorized as VOHC (CDC 2021 ; WHO 2022 ). The B.1.525 (Eta); B.1.526 (Iota); B.1.526.1; B.1.617; B.1.617.1 (Kappa); B.1.617.3; B.1.1.28.2/P.2 (Zeta); B.1.1.28.3/P.3; and B.1.427 and B.1.429 (Epsilon) variants are currently categorized as VUM or as formerly monitored variants, since no clear evidence of their severe phenotypic or epidemiological impact exists (WHO 2022 ). These variants are no longer detected or are circulating at very low levels in the USA and Europe, and as such, do not pose a major significant or imminent risk to Public Health (CDC 2021 ; WHO 2022 ). The Lambda (C.37) and Mu (B.1.621) variants are labelled as VOI and have been under evaluation with respect to their phenotypic features (WHO 2022 ).
The Alpha (B.1.1.7), Beta (B.1.351 and descendent lineages), Gamma (B.1.1.28.1/P.1 and descendent lineages), Delta (B.1.617.2 and descendent lineages), and Omicron (B.1.1.529 lineage along with the BA.1, BA.2 and BA.3 desdencent sublineages) variants are currently labelled as VOC and are responsible for different SARS-CoV-2 phenotypes (CDC 2021 ; Zhou and Wang 2021 ; WHO 2022 ).
These variants have acquired a transmission advantage in comparison to the wild-type virus. In addition, the Omicron variant has been demonstrated to have a replication advantage over the remaining VOCs (CDC 2021 ; McIntosh et al. 2022 ; WHO 2022 ). The SARS-CoV-2 VOCs (specially, Gamma and Delta variants) are suggested to be more pathogenic (resulting in a higher risk of hospitalization and death); and it is reported that a reduction in neutralization by post-vaccination sera and a potential reduction in neutralization by the approved monoclonal antibody treatments against COVID-19 occurs upon SARS-CoV-2 VOC infection (mainly for Gamma, Delta, and Omicron variants) (Davies et al. 2020 ; CDC 2021 ; Khateeb et al. 2021 ; Lopez Bernal et al. 2021 ; Zhou and Wang 2021 ; McIntosh et al. 2022 ; WHO 2022 ). All VOC share the Spike mutation D614G (mutation from aspartic acid to glycine at position 614), which was first identified in March 2020 (Lauring and Hodcroft 2021 ). Considering that the binding of the Spike receptor-binding domain to the ACE2 is essential for SARS-CoV-2 to enter and infect cells, key mutations within the RBD have the potential to modulate the binding ability (Zhou and Wang 2021 ; McIntosh et al. 2022 ). Viruses bearing this mutation have rapidly spread and dominated worldwide; they are suggested to have a more transmissible phenotype with respect to a higher replication capacity and higher levels of viral load in the respiratory tract; and associated to an increased ACE2 binding affinity (Cho and Glenn 2020 ; McIntosh et al. 2022 ).
Transmission of Alpha, Beta, and Gamma VOCs has been significantly reduced over time, and these variants are not widely circulating (CDC 2021 ; McIntosh et al. 2022 ; WHO 2022 ). Omicron VOC has surpassed the Delta VOC as the predominant variant responsible for SARS-CoV-2 transmission, worldwide (ECDC 2022 ; McIntosh et al. 2022 ; WHO 2022 ). While the BA.1 sub-lineage of Omicron has been established as dominant, BA.2 reported sequences have recently increased in proportion in comparison to other Omicron sub-lineages (ECDC 2022 ; WHO 2022 ). Hence, the current epidemiological context with respect to Omicron VOC should be closely monitored.
There is a permanent concern regarding how emerging mutations in the virus may direct it to escape the developed diagnostics and therapeutics, which may consequently reduce the impact of vaccine and drug development efforts (Cho and Glenn 2020 ). A global overview of the SARS-CoV-2 VOC is summarized in Table Table2 2 .
Summary description of the characteristics of SARS-CoV-2 variants of concern 1
1 Adapted from; Zhou and Wang 2021 ; CDC 2021 ; NSW government 2021 ; McIntosh et al. 2022 ; WHO 2022
2 Pango (or Pangolin) lineage: Phylogenetic Assignment of Named Global Outbreak
3 Spike-Receptor-binding domain (RBD) mutations are marked in bold
4 Detected in a limited number of sequenced viruses
Induction of inflammatory cytokines
The interferon-mediated immune response has a prominent role in limiting infection. It is triggered by the host’s perception of pathogen-associated molecular patterns (PAMPs). PAMPs are recognized by the host pattern recognition receptors (PRR). Toll-like receptors (TLRs) and RIG-I-like receptors (RLRs) constitute distinct families of PRR that sense viral nucleic acids, activating transcription factors that lead to the production of type I interferon (IFN-I) (Akira et al. 2006 ; Kawai and Akira 2008 ). There are three main pathways leading to the production of IFN-I. The RIG-I receptor signaling pathway, activated by RNA viruses, the TLR3- and TLR4 TRIF-dependent TLR receptor signaling pathway, and the TLR7/8- and TLR9 MyD88-dependent TLR receptor signaling pathway (Kato et al. 2005 ; Barreiro et al. 2009 ). The activation of these receptors triggers transcription factors, such as NF-κB, the interferon regulatory factors IRF3 and IRF7, and the production of IFN-I. In the extracellular environment, IFN-I reacts with its specific receptor (IFNAR) to trigger the JAK-STAT signaling cascade (Janus kinase-signal transducer and activator of transcription), inducing the phosphorylation of STAT1 and STAT2 and the formation of the STAT1-STAT2 heterodimer. The STAT1-STAT2 heterodimer connects to the IRF9 transcription factor, forming the IFN Stimulated Gene Factor 3 (ISGF3) complex. In the nucleus, ISGF3 binds to the promoter Interferon-Stimulated Response Element (ISRE). This leads to the transcription of IFN-stimulated (ISN) genes, whose effector products promote the antiviral response by modulating the signaling pathways or by exerting direct antiviral activity (Platanias 2005 ; Sadler and Williams 2008 ; Yoneyama and Fujita 2009 ; Schoggins et al. 2011 ). Regulation by these cascades has been identified in CoV infections (Dandekar and Perlman 2005 ; Channappanavar and Perlman 2017 ; Ortiz-Prado et al. 2020 ).
Dissimilarities in IFN-I inhibition between SARS-CoV and SARS-CoV-2 have been reported (Lokugamage et al. 2020 ). SARS-CoV-2 is more sensitive to IFN-I, through phosphorylation of STAT1 and induced ISG proteins, while SARS-CoV is indifferent to its antiviral effects. The differential host innate immune modulation between the two viruses could originate the observed contrasts in disease pathophysiology and progression to severe disease. Investigations using an approach based on the determination of differentially conserved positions (DCPs) affecting viral protein structure and function evidenced changes in viral proteins, between the two epidemic CoV strains, that may affect IFN-I inhibition. An early and properly localized, IFN-I response can effectively limit CoV infection (Rausell et al. 2010 ; Bojkova et al. 2020 ; Trigueiro-Louro et al. 2020 ). Conversely, failure to elicit an early IFN-I response has been associated with severe CoV disease (Channappanavar et al. 2019 ; Hadjadj et al. 2020 ). Moreover, IL-6 and C-reactive protein are significantly upregulated in patients that died, compared to convalescent COVID-19 patients (Lavillegrand et al. 2021 ). High cytokine levels in patients with severe COVID-19, indicating that a storm of chemokines and cytokines occurred could be associated with the severity of the disease (Qin et al. 2020 ). Increased levels of pro-inflammatory cytokines were associated with major pulmonary inflammation and extensive lung damage in both SARS and MERS (Wong et al. 2004 ; Mahallawi et al. 2018 ). A leading cause of COVID-19 mortality is respiratory failure caused by acute respiratory distress syndrome (ARDS). This excessive inflammatory reaction has been considered an important contributor to ARDS and multiple organ dysfunction syndrome (Wang and Ma 2008 ; Suri et al. 2021 ).
Interestingly, studies in human alveolar epithelial cells and macrophages showed SARS-CoV-2 to be a less potent inducer of proinflammatory cytokines than influenza viruses or MERS-CoV. In contrast to influenza virus infection, and as previously observed with MERS-CoV, there appears to be no evidence that the elevated levels of cytokines and chemokines observed in intensive-care unit (ICU) COVID-19 patients, are major contributors to SARS-CoV-2 pathogenesis. It has been suggested that these traits perhaps reflect the more severe lung damage that has taken place (Chan et al. 2013 ; Hui et al. 2020 ; Liu et al. 2020 ).
Furthermore, a suggested consequence of macrophage activation and pro-inflammatory cytokine release could be to trigger a coagulation cascade, leading to immune-mediated arterial thrombosis (Merad and Martin 2020 ). The involvement of TNF and IL-1 cytokines could also imply the activation of cytokine and transcription factor signaling, such as the MAPK and NF-kappa B signaling pathways.
Adaptive immune response
Most viral infections eventually lead to the death of host cells through different types of regulated cell death (RCD) mechanisms (Tang et al. 2019 , 2020 ). CoVs are known to decrease T cell populations, by inducing the intrinsic and extrinsic pathways of T cell apoptosis, thereby compromising B cell activation and humoral immunity (Chu et al. 2016 ; Channappanavar et al. 2019 ).
T cells are one of the major components of the adaptive immune system. They play a vital role in antiviral immunity. Major groups of effector T lymphocyte are differentiated according to the co-receptor (CD8 or CD4) used to bind the human leucocyte antigen (HLA) molecules where viral antigens are loaded. On one hand, to eradicate viruses from the host, the CD8 + cytotoxic T cells kill their target cells primarily by releasing molecules such as perforin, granzymes, and IFN-γ. On the other hand, the role of CD4 + helper T cells includes activating other immune cells, releasing cytokines. The CD4 + helper T cells enhance cytotoxic T cells and B cells, to generate stronger antibody responses and help clear the pathogen.
The role of T cell activation in COVID-19 clinical recovery is incontrovertible. T-cell mediated adaptive immune responses have been shown to be critical in the clearance of CoV infections including SARS-CoV-2 infection in animal models (Zhao et al. 2010 ; Sun et al. 2020 ). In humans, T cell responses are required for protection from clinical disease, contributing to the elimination of the SARS-CoV-2 virus, conferring protective immunity and leading to clinical recovery (Li et al. 2020a ; Nicoli et al. 2020 ). SARS-CoV-2-specific CD4 + and CD8 + T cell responses were observed in many of the patients who had recovered from COVID-19 (Peng et al. 2020 ). Zhao and collaborators concluded that T cells made up one of the three critical axes for viral clearance and disease resolution, along with IFN I and signal transducer and activator of transcription 1 (STAT1) (Zhao et al. 2010 ). T cells are important in immunopathology as well as cross-immunity to other coronaviruses (Zhao et al. 2016 ; Weiskopf et al. 2020 ).
In the early stages of severe COVID-19, patients show a significant reduction in the number of CD4 + T cells, CD8 + T cells, B cells and natural killer (NK) cells, monocytes, eosinophils and basophils (Huang et al. 2020 ; Qin et al. 2020 ; Wu J et al. 2020b ; Xu et al. 2020 ). In patients with acute moderate or severe COVID-19, however, the absolute numbers and relative frequencies of CD4 + and CD8 + T cells are unphysiologically low (He et al. 2020 ; Liu et al. 2020 ; Sekine et al. 2020 ). Several investigations suggest that this is due to the functional exhaustion of T cells that could result from increased expression of programmed cell death (Diao et al. 2020 ; Zheng HY et al. 2020a ; Zheng M et al. 2020b ). Diao and collaborator observed that the numbers of total T cells, CD4 + T and CD8 + T cells are negatively correlated to levels of TNF-α, IL-6, and IL-10, respectively, suggesting these cytokines may be involved in the decrease of T cells detected in COVID-19 (Diao et al. 2020 ).
The genetic basis of COVID-19 susceptibility and severity
From a perspective on the genetic basis of covid-19 susceptibility and severity, researchers are interested in identifying the molecular pathways governing the remarkable range in the clinical presentation of COVID-19. Although in recent assessments some of the new SARS-CoV-2 variants appear to increase disease severity and mortality, there is no evidence that viral mutations in SARS-CoV-2 could explain the extent in which clinical phenotype variation has been observed nor the observed differences in the geographic distribution of COVID-19 (Debnath et al. 2020 ; Pan et al. 2020 ; Zhang et al. 2020a ). Disease outcomes are also affected by age, sex, and stochastic events. Moreover, the immune system ages after each infection and is trained by past infections. Nevertheless, the wide range in the clinical presentations observed suggests that host genetics could also be a major contributor to the differences observed in the host–pathogen relationship.
A wide spectrum of COVID-19 clinical presentations
The spectrum of COVID-19 clinical features ranges from mild disease (none or a mild pneumonia), 81%, severe disease (e.g., with dyspnea, hypoxia, or > 50% lung involvement on imaging within 24 to 48 h), 14%, critical disease (e.g., with respiratory failure, shock, or multiorgan dysfunction), 5%, with an overall case fatality rate of 2.3% (McIntosh et al. 2022 ). Moreover, there remains uncertainty about the proportion of individuals that remain asymptomatic, with a wide range of reports across studies. Pediatric populations aged under 20 years, appear to be less vulnerable to COVID-19 than adults, representing only 2% of the reported cases, with more than 90% of the cases either being asymptomatic, mild, or moderate. Interestingly, the emerging multisystem inflammatory syndrome in children (MIS-C), or the COVID-19 associated pediatric inflammatory multisystem syndrome temporally associated with SARS-CoV-2 (PIMS-TS), are multisystem inflammatory conditions that overlap with the diagnostic criteria of Kawasaki disease (KD) and KD shock syndrome (Jiang et al. 2020 ).
Population-based genotype to phenotype association studies
Genetic variants affecting clinical phenotype can be identified through genotype to phenotype association studies. Once identified, genetic determinants of disease susceptibility and severity can contribute to disease control as a tool in early diagnosis for risk evaluation, allowing patients at greater risk to be prioritized for protective prophylactic vaccination or to be referenced to special medical care and surveillance. Moreover, genetic association studies have been central to the development of novel therapeutic schemes aimed at surpassing deficiencies in gene products with causal implications in severe disease (Holland 2001 ; Alangari et al. 2011 ; Casanova et al. 2014 ). This is an ongoing strategy in COVID-19 investigations (Bastard et al. 2020 ; Zhang Q et al. 2020b ).
The genome-wide association study (GWAS), also known as the whole genome association study (WGAS), and the candidate gene association study (CGAS) are population-based approaches in genotype to phenotype association. A GWAS is an untargeted hypothesis-free genome-wide screening strategy. It is the usual approach for common variants. The candidate gene approach is a rational approach where the targeted gene/s and variant/s are selected based on a priori hypothesis about their implication in disease causation (Fig. 1 ) (David 2021 ). It is more adaptable to identify lower minor allele frequency variants than the GWAS and may also be considered in validation strategies for genome-wide studies. The availability of cost effective next-generation sequencing (NGS) resources has been key to the gained momentum in the CGAS approach.

Critical steps to CGAS: scientific protocol, study design, phenotype definition, candidate gene and variant selection, haplotype analysis and linkage disequilibrium (LD), sample size estimation, statistical significance and p -value, exploratory data analysis (EDA) and quality control of genotyping data, testing statistical association and functional analysis (adapted from, David 2021 )
In simplified terms, the GWAS can be viewed as hypothesis-generating and the CGAS as hypothesis-testing approaches (Fig. 2 ).

To support a causal association, case–control genetic association studies can be validated through replication. Candidate gene association studies in targeted biological pathways are useful for the replication of genome-wide results. They are also more adaptable to identify lower minor allele frequency variants and thus more prone to a functional analysis follow-up. Meta-analysis is a statistical method for analyzing large collections of results from independent association studies including candidate gene and genome-wide association studies. Meta-analysis can be used to compile results from methodologically uniform studies (conventional application) (Skol et al. 2006 ; Zondervan and Cardon 2007 ) or from various methodological approaches (Li et al. 2020c ; Pairo-Castineira et al. 2021 ; Parkinson et al. 2020 ). Meta-analysis of genome-wide overlap (MAGO), or Meta-analysis of overlapping SNVs from genome-wide approaches, provides a relevant means of aggregating evidence for causal association. There is an increased need for replication strategies by which to infer causality for candidate gene selection and validation, pointing to the possible role of other candidate pathway-related targets
Genome-wide and candidate gene association studies applied to COVID-19
Of interest to genome-wide and candidate gene association studies, the current genetic theory of infectious diseases, suggests a monogenic (single gene) basis of most infections, characterized by incomplete penetrance (non-Mendelian) and genetic heterogeneity (multiple genes and alleles) but physiological homogeneity (a molecular basis pointing to a cellular and immunological mechanism) (Casanova and Abel 2020 , 2021 ). Applied to today’s pandemic, most humans would, in fact, be “immunodeficient” with respect to SARS-CoV-2 and a physiological homogeneity in the molecular pathways governing severe COVID-19 is to be expected.
Whole-genome studies and whole-exome sequencing (WES) have taken the lead in the efforts to identify the human genetic determinants of COVID-19 susceptibility and severity. Several consortiums are now contending with this task including the COVID Human Genetic Effort ( https://www.Covidhge.com/ ) (Casanova et al. 2020 ), deCODE Genetics ( https://www.decode.com/ ) (Hakonarson et al. 2003 ), the COVID-19-GR ( http://www.gsrt.gr/central.aspx?sId=119I428I1089I646I488772 ) and the Harvard’s Wyss Institute and the Personal Genome Project at Harvard University ( https://wyss.harvard.edu ). In particular, the COVID-19 host genetics initiative provides an environment to foster the sharing of resources to facilitate COVID-19 host genetics research (e.g., protocols, questionnaires), organizes analytical activities across the studies, and provides a platform to share results to the benefit of the broader scientific community (COVID-19 Host Genetics Initiative 2020 ).
From a different perspective, in a new disease like COVID-19, for which the pathophysiological mechanisms of the disease remain largely unknown, the rational approach of a CGAS poses an obvious challenge. Hypothesis generating for candidate gene studies of COVID-19 relies on our knowledge of the prepandemic CoV infections, our current understanding of the molecular mechanisms governing the pathophysiology of SARS-CoV-2 infection, and recent findings from genome wide studies. Since the beginning of the pandemic, several reviews have been published on the host genetics of the immune response associated with COVID-19 susceptibility, severity, and outcome (Table (Table3) 3 ) (Anastassopoulou et al. 2020 ; Carter-Timofte et al. 2020 ; Elhabyan et al. 2020 ; Godri Pollitt et al. 2020 ; LoPresti et al. 2020 ; Oladejo et al. 2020 ; Ovsyannikova et al. 2020 ; Zunec 2020 ; Colona et al. 2021a ; SeyedAlinaghi et al. 2021 ; Suh et al. 2022 ). Moreover, in line with this renewed interest, in a recent systematic review of genes related to viral susceptibility reported in human genetic studies, the CGAS approach was considered as a valid approach (Elhabyan et al. 2020 ).
Review articles identifying candidate genes through COVID-19 host-related factors
1 Systematic review inquiry: "Genetic Predisposition to Disease"[Majr] AND "Virus Diseases"[Majr]; "SARS Virus"[Mesh] AND Genetic [Title/Abstract]
2 Systematic review inquiry: “SARS-CoV-2,” “2019- nCoV,” AND “COVID-19”; “polymorphisms,” “allelic variation,” “genetic predisposition,” “genotype,” “clinical outcome”; names of individual genes in which relevant polymorphisms were found
3 Systematic review inquiry: diferent combinations of keywords in the following orders, 1. “COVID-19” or “SARS-CoV-2” or “Novel Coronavirus” or “2019-nCoV” or “Coronavirus” (title/abstract); 2. “Genetic susceptibility” or “Genetic vulnerability” or “Genetic probability” (title/abstract); 3. (A) and (B)
4 Systematic review inquiry: ((SARS-CoV-2[tiab] OR COVID-19[tiab] OR “Coronavirus disease”[tiab] OR “Severe acute respiratory syndrome coronavirus 2”[tiab] OR coronavirus[tiab]) OR (SARS-CoV2[Mesh] OR “Spike Glycoprotein, Coronavirus”[Mesh] OR COVID-19[Mesh] OR Betacoronavirus[Mesh] OR “Coronavirus Infections”[Mesh])) AND ((“Variant gene”[tiab] OR “whole-exome sequencing”[tiab] OR “allele frequency”[tiab] OR mutations[tiab] OR “protein–protein interaction”[tiab] OR “Significant linkage disequilibrium”[tiab] OR LD[tiab] OR PPI[tiab] OR Variants[tiab] OR Coding[tiab] OR Missense[tiab] OR “epigenetic modifcation”[tiab] OR polymorphism[tiab]) OR (“Molecular Docking Simulation”[Mesh] OR “Protein Interaction Domains and Motifs”[Mesh] OR “Virus Internalization”[Mesh] OR “High-Troughput Nucleotide Sequencing”[Mesh] OR “Polymorphism, Single Nucleotide*”[Mesh] OR “Real-Time Polymerase Chain Reaction”[Mesh]))
Considerations on the definition of clinical phenotype
In genetic association studies, a clear definition of the clinical phenotype is key, for it must be kept in mind that different definitions of phenotype typically lead to different results (Fig. 3 ). The need for respiratory support or death due to COVID-19 are major criteria for a severe phenotype classification of laboratory confirmed SARS-CoV-2 infection (Table (Table4). 4 ). The main strategy undertaken by the consortium COVID Human Genetic Effort is to analyze previously healthy young patients with severe and unexplained forms of COVID-19 (Casanova et al. 2020 ). A second strategy adopted by this group intends to explain why some individuals, despite heavy exposure to SARS-CoV-2, remain healthy and seronegative, particularly those individuals that also test negative for T cell responses to SARS-CoV-2, that is, they appear to be naturally resistant to SARS-CoV-2 infection.

Illustration of different definitions of phenotype leading up to different results in case–control genetic association studies
Phenotypic criteria for genetic association studies of COVID-19
Adapted from COVID-19 Host Genetics Initiative 2020 ( https://www.covid19hg.org/about/ ) and COVID Human Genetic Effort 2020 ( https://www.Covidhge.com/ )
A detailed list of criteria for phenotype definition of severe COVID-19 is given in the COVID-19 Host Genetics Initiative website (COVID- 19 Host Genetics Initiative 2020 ). It includes demographic data, risk factors, symptoms at admission Moreover, care and hospital related attributes and possible comorbidities of the immune, cardiovascular, neurological, and respiratory systems, as well as cancer, taken into account, also contribute to improve the reproducibility of genetic association studies. Male sex was identified by a global COVID-19 meta-analysis as being a risk factor for death and ITU admission (Peckham et al. 2020 ). This observation may be due to sex differences in both the innate and adaptive immune systems or sex differences in X chromosome linked ACE2 receptor expression (Peckham et al. 2020 ). Moreover, significant positive genetic correlations were detected for several adiposity phenotypes (Pairo-Castineira et al. 2021 ). Vitamin D status has also been recently recognized risk factor for COVID-19, with deficiency conferring greater risk (Grant et al. 2020 ). Other possible cofactors of disease severity include secondary infections and quantitative traits that have been associated with disease severity such as mannose-binding lectin (MBL), ferritin, D-dimer, C-reactive protein, and increased levels of proinflammatory cytokines, absolute neutrophil counts, and the neutrophil to lymphocyte ratio (NLR) (Ortiz-Prado et al. 2020 ; Ovsyannikova et al. 2020 ).
Causality in genotype to phenotype associations
To support a causal association of the alleles identified, case–control genetic association studies need to be validated: Validated results are core in tracking other pathway related targets with increased advantages, such as druggability (Baillie 2014 ; King et al. 2019 ). In the case of COVID-19, the selection of possibly more druggable targets is strategic (Parkinson et al. 2020 ; Novelli et al. 2021 ). The validation process is elaborate and begins as early in the process as the initial design of the scientific protocol, from the careful and correct definition of phenotype to in vitro and in vivo validation through functional anylisis (Fig. 1 ) (David 2021 ). Other points for CGAS and WGAS are discussed below.
Replication
One means of validation genotype to phenotype associations is through replication (Fig. 2 ). There is an increased need for replication strategies by which to infer causality for candidate gene selection and validation. Conventionally, a replication study involves the analysis of the same variants in the same direction of effect and in the same (ethnic) population, considering the same phenotype as the original study (Skol et al. 2006 ; Zondervan and Cardon 2007 ). However, the findings can also be replicated in different populations, using different study designs, or applying various methodological approaches. Conformingly, the use of meta-analysis (MA), as an element of a systematic review, is a statistical method of analyzing large collection of results from independent CGAS or genome-wide association studies. Data from several independent CGAS can be grouped by meta-analysis, for power and precision increment in the association results. Furthermore, meta-analysis of overlapping single nucleotide variants (SNVs) from genome-wide approaches, or Meta-analysis of genome-wide overlap (MAGO), provides a relevant means of aggregating evidence for causal association within and across genome-wide methodological approaches.
Criticalities of GWAS
Although the contribution of GWAS to the understanding of the genetic determinants of disease is widely accepted, several caveats to the validation of GWAS results exist (Colona et al. 2021a , 2021b ). These include the facts that (1) the effect sizes of the identified alleles, namely in terms of odds ratio (OR), are generally too low (OR < 2) for these to be considered as predictive genomic markers (Table (Table5); 5 ); (2) most of these studies do not take into account the contribution of nongenetic and environmental factors implicated in the disease phenotype; (3) many of the biobanks currently available lack essential complementary patient information and are unrepresentative of mixed and complex ethnic groups; (4) computer algorithms can lead to interpretive errors if they are based on representative alleles from international reference databases and certain predictive functional bioinformatics characteristics; (5) studies based on sampling hospitalized patients and the general population as controls for which no information is available about exposure to the virus might lead to an overstatement of the results and collider bias.
MAIC score rank of some candidate genes from cited references and respective biological pathways as included in KEGG 2019 (Human)
Chr. loc. chromosome location; NA not available; NR not reported in top 2000 MAIC score rankings; OR odds ratio
1 MAIC score rank, within a range of 2000, version April 14, 2021 ( https://baillielab.net/maic/covid19 , accessed June 18, 2021)
2 Risk estimates–Odds ratio (OR)
3 TLR3, UNC93B1, TICAM1, TBK1, IRF3, IRF7, IFNAR1, IFNAR2 (autosomal-dominant model–ADM) with risk estimates (odds ratios) of 9 (Zhang et al. 2020b )
4 IRF7, IFNAR1 (autosomal-recessive model–ARM) with risk estimates (odds ratios) > 50 (Zhang Q et al. 2020b )
Novel approaches to circumvent these shortcomings play a major part in the current efforts of the scientific community (Colona et al. 2021b ; Moon et al. 2021 ). Largely the need for more holistic approaches is recognized. These may call upon phenome-wide association study (PheWAS) approaches as well as the use of functional genomic databases (Colona et al. 2021b ). The phenome-wide association study (PheWAS) approach is a complementary approach that can use genetic pleiotropy to validate known comorbidities and provide insight into biological and molecular mechanisms (Hebbring 2014 ; Moon et al. 2021 ). Moreover, functional genomic databases such as the Genotype-Tissue Expression (GTEx) database, grouping genotyping data linked to genome-wide gene expression patterns across a wide range of tissue types, allow for analysis at tissue-specific resolution ( https://www.gtexportal.org ) (Lonsdale et al. 2013 ).
Candidate genes and pathways associated with COVID-19
Recently, several genotype to phenotype association approaches have been deployed, contributing to the identification of candidate genes governing COVID-19 susceptibility and severity. These include in silico approaches; candidate gene approaches based on knowledge of the pathophysiological mechanisms of common human viral infections, COVID-19 and of other prepandemic CoV infections; and genome wide approaches such as GWAS, transcriptomics and CRISPR-Cas9 knockout screens.
In silico approaches
The human leukocyte antigen (hla) system.
The human leukocyte antigen (HLA) system is intrinsically implicated in an effective T cell mediated immune response. A central part of anti-viral immunity is antigen presentation to CD4 + and CD8 + T cells by the HLA system. HLA class I and HLA class II are cell-surface glycoproteins that regulate the immune system by presenting foreign peptides to T cells. In the case of viral epitopes, these are presented by HLA class I molecules to CD8 + T lymphocytes and HLA class II molecules to CD4 + T lymphocytes which can assist antibody-producing B-cells.
The HLA genes have come into focus as a potential marker for COVID-19 susceptibility and severity (Li et al. 2020a ; Zunec 2020 ). Allele and haplotype frequencies in class I and class II HLA molecules have been associated with either protective or deleterious effects to MERS and SARS-CoV. Although, it has been difficult to detect a clear pattern of association due to the extreme polymorphic nature and extraordinary variation in the geographic distribution of the alleles, in silico approaches can contribute to the overall strategy of identifying target HLA polymorphisms for CGAS. As a best option for CGAS, HLA variants should be analyzed at the allelic level (several linked SNVs) and not at the SNV level. Nguyen and collaborators, in a comprehensive in silico analysis of the binding affinity of SARS-CoV-2 peptides across multiple HLA genotypes, identified HLA-B*46:01 as having the fewest predicted binding peptides for SARS-CoV-2. Based on this finding, the authors suggested its association with higher vulnerability to COVID19. On the contrary, HLA-B*15:03, having the greatest capacity to present highly conserved SARS-CoV-2 peptides that are shared among common human coronaviruses, could suggest a potential cross-protective T cell immunity (Nguyen et al. 2020 ). Recognizably, large, and reproducible studies are needed to circumvent the polymorphic nature of these genes. To surpass these shortcomings, Weiner 3 rd and collaborators using HLA sequencing through a combined a multicentric approach with individuals from several countries, included covariates aimed at excluding potential confounding effects, then tested the association results by meta-analysing data from prior genome-wide association studies (GWAS) and combined the analysis with results of in silico epitope modeling (Weiner 3 rd et al. 2021 ). These authors identified HLA-C*04:01 as a risk factor for severe COVID-19 with an OR of 3.5 (Weiner 3 rd et al. 2021 ).
The ACE2 SARS-CoV-2 receptor
Resistance to infections has been associated with the lack of a receptor for the pathogen (Casanova and Abel 2020 , 2021 ). The human ACE2 protein is the recognized receptor for SARS-CoV-2 in human cells. ACE2 SNVs, have been suggested as potential evidence for a genetic basis underlying variable geographic distributions of COVID19 in human populations . Using a structural modeling approach, Hussain and collaborators investigated how the binding of the SARS‐CoV‐2 spike protein with proteins encoded by different human ACE2 allelic variants could contribute to the susceptibility and/ or resistance against the viral infection (Hussain et al. 2020 ). The authors identified two ACE2 alleles that may provide potential resistance to SARS-CoV-2 infection, rs73635825 (S19P) and rs143936283 (E329G). In another study, based on the analysis of a large WES and molecular dynamic analysis simulations of protein structural changes caused by a small number of selected missense variants, Benetti and colleagues concluded that ACE2 is one of the main molecules whose genetic heterogeneity can modulate infection and disease progression (Benetti et al. 2020 ). Other studies also identified ACE2 variants affecting the binding affinities for the S protein, on the premise that these could influence COVID-19 susceptibility (Li et al. 2020b ; Suryamohan et al. 2021 ). Interestingly, in a recent review on the SNVs affecting COVID-19 severity, ACE2 , along with TMPRSS2 , a protease coding gene, and IFITM3 , an interferon induced antiviral protein gene, were the genes most mentioned as related to SARS-CoV-2 infection (Suh et al. 2022 ).
Candidate gene approaches
Relying on our current knowledge of the immune response to SARS-COV-2 and examples offered by other human viral infections can guide researchers towards hypothesis driven research on the genetic determinants of COVID-19.
The genes that encode the proteins involved in SARS-CoV-2 entry into the host cells are major targets. The prominent role of proteases during the initial stages of SARS-CoV-2 cell entry, points to the endosomal and non-endosomal proteolytic pathways. Based on our current understanding, candidate genes encoding proteases include the CTSL , TMPRSS2 , PCSK3/ FURIN , and HAT genes. Considering the critical function of the respiratory tract in the initial contact with the virus, Novelli and collaborators performed a pilot-study aimed to verify, in nasopharyngeal and oropharyngeal samples, the in situ expression of predicted SARS-CoV-2 host invasion genes ( ACE2, TMPRSS2, PCSK3/ FURIN, EMILIN1, EMILIN2, MMRN1, MMRN2, DPP4, GAPDH, RPLP0, ACTB ) (Amati et al 2020 ). These genes were subsequently sequenced; identifying genetic variants associated with COVID-19 in the TMPRSS2 and PCSK3/FURIN genes (Latini et al. 2020 ). In a similar sequential approach joining in situ expression of candidate genes, sequencing and functional studies, but directed to a later phase of RNA viruses (cell egression), this group identified rare germline activating variants associated with severe COVID-19 cases in the NEDD4 and WWP1 genes of the HECT-E3 ligase family, (Novelli et al. 2021 ).
In the initial stages of viral infection, hyperactivation of cofilin and inefficient actin polymerization is known to occur (Godri Pollitt et al. 2020 ). The possible implications in the clinical phenotype of COVID-19 has been suggested for variants in the DSTN gene, which encodes the actin-depolymerizing factor (ADF), and in the genes encoding cofilin, CFL1 and CFL2 .
The IFN-I response is pivotal to the infectious process of the influenza virus as well as it appears to be for the SARS-CoV-2 infection. The signaling cascades involved, from the recognition of the viral particles by host PRRs to the transcription of ISGs, contain an abundance of candidate genes. Notably the intracellular Toll-like receptors (TLRs), such as TLR3, TLR7, TLR8, and TLR9, are particularly relevant for viral recognition as supported by clinical genetics data and the evidence of strong selective pressure exerted on these genes (Barreiro et al. 2009 ). Moreover, acting on the premise that influenza virus infection is a possible co-factor of severe COVID-19, and that a deficient IFN-I response appears associated with severe disease in both of these viral infections (Ciancanelli et al. 2015 ; Chen et al. 2018 ), it appears relevant to observe the similarities in the immune response to the viruses and explore the possibility of genetic determinants in the signaling cross-talk.
In one study, rare putative loss-of-function variants, recently identified in the TLR7 , associated with impaired type I and II IFN responses of 4 young male patients with severe COVID19 (Van Der Made et al. 2020 ).
The most compelling understanding for the successful application of a CGAS approach to the task of identifying reproducible disease gene associations in COVID-19, however, resulted from a recent study by Zhang and collaborators on the IFN pathway genes (Zhang et al. 2020b ). A NGS technology was used to investigate 13 candidate genes implicated in life-threatening influenza and other viral illnesses ( TLR3 , IRF7 , and IRF9 ) and core genes conferring influenza susceptibility ( TICAM1/ TRIF , UNC93B1 , TRAF3 , TBK1 , IRF3 , and NEMO/ IKBKG from the TLR3-dependent type I IFN induction pathway, and IFNAR1, IFNAR2, STAT1 , and STAT2 from the IRF7- and IRF9-dependent type I IFN amplification pathway) (Zhang et al. 2020b ). From this study it appeared that rare coding variants of 8 of these candidate genes ( TLR3 , UNC93B1 , TICAM1 , TBK1 , IRF3 , IRF7 , IFNAR1 , IFNAR2 ) accounted for about 3% of critical COVID-19 cases, with risk estimates (odds ratios) of 9 (Table (Table5). 5 ). These results evidenced the essential role of type I IFNs in the protective immune response against SARS-CoV-2 infection. They pointed to the possible role of the > 400 other candidate type I IFN-related genes. Finally, they incited investigations hypothesizing that neutralizing auto-antibodies (auto-Abs) against type I IFNs might also underlie life-threatening COVID-19 pneumonia. A subsequent study led to the demonstration that auto-Abs against type I IFNs account for at least 10% of critical cases (Bastard et al. 2020 ).
Downstream from the recognition of viral particles by the PRR, innate response cytokines determine the clinical course of the disease. An exacerbated pro-inflammatory cytokine response may lead to the development of ARDS. These observations and many others provide evidence of the importance of the PRRs, the specific cytokines and their pathways including their receptors and effectors, relating to a myriad of potential candidate genes for genetic association studies.
In COVID-19, both the intrinsic and extrinsic pathway of cell death by apoptosis are activated during later stages of the disease. These are highly dependent on the activity of cysteine proteases of the caspase group. The investigation of these proteases, as well as the CTSs, would also be warranted due to the roles that these proteins play in regulating cell death mechanisms (Chwieralski et al. 2006 ; Minarowska et al. 2007 ; Schrader et al. 2010 ; Repnik et al. 2012 ; De Castro et al. 2016 ).
Genome-wide approaches
Genome-wide approaches are relevant to the identification of common variants associated with disease. Although the identified variants may be common in the population and their allelic variability confers only a small but detectable effect on the disease phenotype, they can underline the key molecular pathways implicated in severe disease. Stoeger and Amaral, revising candidate genes in COVID-19 publications, flag that research into human protein-coding genes is disproportionately skewed towards a comparably small set of genes, and that genes that are identified by genome-wide datasets, and hence likely to have biological significance in the context of COVID-19, are at a risk of remaining ignored by researchers (Stoeger and Amaral 2020 ).
GWAS approaches as a source of candidate genes
In collaboration with the Covid-19 Host Genetics Initiative, the Severe Covid-19 GWAS Group, in a large GWAS, identified, in Spanish and Italian populations, two genomic loci associated with severe Covid-19 (Ellinghaus et al. 2020 ). In the study, severe Covid-19 was defined as hospitalization with respiratory failure and a confirmed SARS-CoV-2 viral RNA polymerase-chain-reaction (PCR) test. The control group was mainly comprised of persons with unknown SARS-CoV-2 infection status, whereas a small number of participants had evidence of the development of anti–SARS-CoV-2 antibodies. Resulting from the choice of the clinical phenotypes, this investigation provided targets associated with COVID-19 respiratory failure as well as COVID-19 susceptibility per se. Candidate genes were identified from the ABO blood group locus at 9q34.2 and from a multigene locus at 3p21.31. A blood-group–specific analysis showed a higher risk in blood group A than in other blood groups and a protective effect in blood group O. This association has also been reported in other studies (Golinelli et al. 2020 ; Zhao J et al. 2021 ). Additionally, at locus 3p21.31, six candidate genes were identified ( SLC6A20 , LZTFL1 , CCR9 , FYCO1 , CXCR6 , and XCR1 ). These findings grounded new directions, and represented a major leap, in identifying the molecular mechanisms governing the pathophysiology of COVID-19 and the determinants of severe disease. The identified association with the chromosomal region 3p21.31 was replicated in association to critical COVID-19 disease in the GWAS performed by Pairo-Castineira and collaborators (Pairo-Castineira et al. 2021 ).
A GWAS approach also identified novel candidate genes and risk variants by examining deaths in participants in UK Biobank testing positive for the SARS-CoV-2. These genes include ALOXE3, TMEM181, BRF2, ERAP2 , and ApoE (Kuo et al. 2020 ; Lu et al. 2020 ).
A targeted analysis of whole-exome sequencing (WES) data evidenced a mutational enrichment of common variable immunodeficiency (CVID) associated genes in severe COVID-19 patients, in the TNFRSF13C gene, encoding the B cell-activating factor receptor (OR = 12.3) (Russo et al. 2021 ).
Genome-wide CRISPR-Cas9 knockout screens as a source of candidate genes
Genome-wide CRISPR-Cas9 knockout screens contribute to the elucidation of genotype to phenotype relationships by ablating gene expression on a genome-wide scale and studying the resulting phenotypic alterations. Several studies have used CRISPR loss-of-function screens to generate functional catalogues of host factors required for infection by SARS-CoV-2 (Baggen et al. 2021 ; Daniloski et al. 2021 ; Schneider et al. 2021 ; Wang et al. 2021 ; Wei et al. 2021 ; Zhu et al. 2021 ). These studies have highlighted the critical pathways and the importance of multiple genes within each pathway. Notable candidate host factors include genes involved in phosphatidylinositol biosynthesis, cholesterol homeostasis, regulation of intracellular transport, regulation of autophagy, and endosomal trafficking.
Transcriptomics
Transcriptomics and expression quantitative trait loci (eQTLs), that associate genomic and transcriptomic data sets are observations that present additional opportunity for candidate gene discovery for associations (Nica and Dermitzakis 2013 ; Pairo-Castineira et al. 2021 ).
The variation in ACE2 expression level may be associated to susceptibility and clinical phenotype of SARS-CoV infection (Hofmann et al. 2005 ). The finding however remains controversial (Butler-Laporte et al. 2021 ). Based on this premise Cao and collaborators, using the GTEx database for eQTL analysis, revealed variable ACE2 allele frequencies across different populations (Cao et al. 2020 ). The effect of ACE2 polymorphisms and expression levels on increased morbidity and lethality of COVID-19 in males has also been speculatively associated with its location on the X chromosome (Liao et al. 2005 ; Gadi et al. 2020 ; Devaux et al. 2020 ; Van Der Made et al. 2020 ). In a genome-wide eQTL analysis, based on high-throughput RNA sequencing, Ansari and collaborators discovered that polymorphisms in the interferon lambda (IFNL) region are associated with ACE2 expression, suggesting the negative correlation between the interferon response and ACE2 expression (Ansari et al. 2020 ). This result could also suggest a possible epistatic relationship between the genetic variants in these genes that also warrants further exploration.
TMPRSS2 levels and genetic variants proved to be possible candidate disease modulators, contributing to the observed epidemiological data among Italian patients (Asselta et al. 2020 ). 149 A haplotype characterized by 3 SNVs (rs2070788, rs9974589, rs7364083), whose MAF is significantly increased in Europeans in respect to East Asians, was predicted to be associated with higher TMPRSS2 expression. The rs2070788 SNV has also been associated with susceptibility to influenza (Cheng et al. 2015 ). Recently a common TMPRSS2 SNV (rs12329760) was associated with a reduced likelihood of developing severe COVID-19 was identified (OR 0.87) (David et al. 2022 ).
Meta-analysis of genome-wide overlap
Meta-analysis of genome-wide association studies are resulting from the plethora of data generated since the onset of the pandemic. In particular, two of the recent publications are central to MAGO applications in the validation of candidate genes in COVID-19 (Parkinson et al. 2020 ; Pairo-Castineira et al. 2021 ). These include an application of the meta-analysis by information content (MAIC) algorithm (Parkinson et al. 2020 ), and a GWAS followed by multiple genome-wide replication approaches (Pairo-Castineira et al. 2021 ).
The MAIC algorithm was originally developed to identify host genes necessary for Influenza A virus (IAV) replication (Li et al. 2020c ). Parkinson and collaborators applied MAIC to identify potential therapeutic targets in COVID-19 (Parkinson et al. 2020 ). In this approach, it is assumed that (1) there exists a set of true positives: host genes involved in COVID-19 pathogenesis; (2) a gene is more likely to be a true positive if it is found in multiple experiments; (3) a gene is more likely to be a true positive if it occurs in a list containing a higher proportion of genes with supporting evidence from multiple sources; (4) due to experimental biases, the evidence that a gene is a true positive is further increased if it is found across experimental types. With these assumptions, MAIC allows the quantification of the information content in a gene list by comparing that list to the results from other experiments, both within and between methodologies, producing a weighting factor for each experiment used to calculate a score for each gene.
For the COVID-19 MAIC application a systematic literature search of PubMed was carried out for: (“Coronavirus” OR “Severe Acute Respiratory Syndrome” OR “Middle East Respiratory Syndrome” OR “Sars-CoV-2” OR “COVID-19”) AND ((gene*.[Title/Abstract]) OR (genom*[Title/Abstract]) OR (transcript*[Title/Abstract]) OR (protein*[Title/Abstract]) OR (“Susceptibility”[Title/Abstract]) OR (siRNA[All Fields])). The methodological types included were CRISPR screens, RNAi screens, Protein–protein interaction e.g. yeast-2-hybrid screens, Host proteins incorporated into virion or virus like particle, Genetic Association Studies, Transcriptomics e.g. RNA-Seq, scRNA-Seq, Proteomic studies e.g. mass-spectrometry, and Selected gene set screens. Candidate-gene human genetic studies were excluded. A final ranked list of genes based on the MAIC score was provided and updated results are made available at https://baillielab.net/maic/covid19 (accessed June 18, 2021). MAIC score distributions of top enriched pathways from KEGG 2019 (Human) Cytokine-cytokine receptor interaction, Influenza A, Hepatitis C, IL-17 signaling pathway, Toll-like receptor signaling pathway, T-cell receptor signaling pathway, MAPK signaling pathway, Apoptosis, Protein processing in endoplasmic reticulum, Antigen processing and presentation. From WikiPathways 2019 (Human), these included Senescence and autophagy in cancer, Toll-like receptor signaling pathway, T-cell antigen receptor signaling pathway, Human complement system, VEGFA-VEGFE2 signaling pathway, Glycolysis and gluconeogenesis, TSLP signaling pathway, IL-3 signaling pathway, Fas ligand pathway/HSP regulation, MAPK signaling pathway.
The MAIC score rank of some candidate genes from references cited in this review are given in Table Table5. 5 . Some of these genes are currently recognized as belonging to the Coronavirus disease—COVID-19 viral infectious disease pathway included in the KEGG PATHWAY Database (Kanehisa and Goto 2000 ). ( https://www.genome.jp/kegg/pathway.html , accessed July 1, 2021), such as IFNAR2, TBK1, TLR3, ACE2, IRF3, IFNAR1, TYK2 . Several of these genes are also implicated in the pathways of other viral infectious diseases such as Herpes simplex virus 1 infection, Hepatitis B, Hepatitis C, the Human immunodeficiency virus 1, and Influenza A. A large number of these genes code for proteins that act upon the immune system and some are implicated in signaling and interaction, and signal transduction.
The MAIC score rankings of a gene appear to vary considerably from one observation to another of the dataset. There is yet no indication on the possibility of a stabilization of the gene rankings as the number of integrated studies increases. This advocates the need for further development of this or other meta-analytical algorithms. Moreover, a failing of the MAIC is that it misses important functional studies (Novelli et al. 2021 ).
In the study presented by Pairo-Castineira and collaborators, MAGO was applied to results replicated from GWAS, Mendelian randomization of gene expression, Transcriptome-wide association study (TWAS) and MAIC analysis (Pairo-Castineira et al. 2021 ). The GWAS was performed in cases of European descent from GenOMICC and a control population from the UK Biobank. Results from the primary analysis were replicated, in cases and controls, in a meta-analysis of data from other data sources (the COVID-19 Host Genetics Initiative 23andMe Inc). Meta-analysis identified overlapping SNVs from six genomic loci: LZTFL1 , CCHCR1 , OAS1–OAS3 , DPP9 , TYK2 , IFNAR2 . Mendelian randomization of gene expression evidenced a causal relationship on the odds of patients developing severe COVID-19: 1) of genes selected a priori as potential therapeutic targets for COVID-19 and; 2) transcriptome-wide. Results were significant for two genes TYK2 , IFNAR2. TWAS was used to link GWAS results to tissue-specific gene expression data by inferring gene expression from known eQTL in whole blood and lung samples from the GTEx v.8 data base ( https://gtexportal.org/home/ ). A meta-TWAS analysis was performed (Barbeira et al. 2018 ). The genes identified with significant differences in predicted expression compared to control individuals were CXCR6 , OAS3 , CCR3 , MAT2B , CCR2 , TNFSF15 , ICAM5 , FYCO1 . The MAIC algorithm was used to analyze these results according to the data-driven gene list weightings to produce a comprehensive ranked list of host genes associated with COVID-19 (Parkinson et al. 2020 ). TWAS revealed the greater overlap of results as compared to that of other MAIC data sources of host genes implicated in COVID-19.
A GenOMICC study was again performed in a more recente whole genome sequencing approach. Critical COVID-19-associated variants were identified, including in genes involved in interferon signalling ( IL10RB , PLSCR1 ), leucocyte differentiation ( BCL11A ), and blood type antigen secretor status ( FUT2 ) (Kousathanas et al. 2022 ). Moreover, in the subsequent TWAS study, using gene expression data for disease-relevant tissues, lung and whole blood, the authors also found evidence implicating multiple genes in critical COVID-19.
The COVID-19 Host Genetics Initiative network recently published the results of three genome-wide association meta-analyses comprising up to 49,562 COVID-19 patients from 46 studies across 19 countries worldwide (COVID-19 Host Genetics Initiative 2021 ). Here, conventional case–control meta-analyses was performed in three main categories of COVID-19 disease phenotype according to predefined and partially overlapping phenotypic criteria: (1) critically ill COVID-19 cases defined as those who required in hospital respiratory support or who were deceased due to the disease, (2) cases with moderate or severe COVID-19 defined as those hospitalized due to symptoms associated with the infection, and (3) all cases with reported SARS-CoV-2 infection with or without symptoms of any severity. Controls for all three analyses were selected as genetic ancestry matched samples generally without known SARS-CoV-2 infection. Thirteen genome-wide significant loci associated with SARS-CoV-2 infection or severe COVID-19 were reported. Four loci were associated to susceptibility to SARS-CoV-2 infection, but not with the progression to more severe COVID-19 phenotypes. These included the previously reported ABO locus. In contrast, the remaining 9 loci were associated with increased risk of severe symptoms, many of which did not ranked within the MAIC top 2000 scores ( https://baillielab.net/maic/covid19/ , version April 14, 2021). Smoking and body mass index were identified as causal risk factors for severe COVID-19.
Interactome studies
Along with GWAS, candidate gene and transcriptome approaches, interactome studies of physical interactions among molecules such as protein–protein interactions (PPIs) between SARS-CoV-2 and human cells can reveal underlying mechanisms of pathogenesis and assist in development of antiviral therapies. In his article, Lee describes the construct of protein sequence-based multi-label classifiers to predict virus-human PPIs with different evidence or confidence levels for SARS-CoV-2 infection (Lee 2021 ). The model also predicts evidence levels for all PPI pairs between the SARS-CoV-2 and human proteomes to provide a draft virus-host interactome landscape of SARS-CoV-2 infection in humans in silico. Finally, it prioritizes those virus-human PPIs or sub-networks of high evidence for biological relevance and therapeutic opportunities for COVID-19 in future studies. Preliminary observations concluded that most human proteins appear to interact with SARS-CoV-2 Nsp7, Nsp1, and ORF14, with significant enrichment in the top 2 pathways of vascular smooth muscle contraction (CALD1, NPR2, CALML3) and Myc targets (CBX3, PES1). It is also suggested that histone H2A components are targeted by multiple SARS-CoV-2 proteins (Lee 2021 ).
The overwhelming impact that COVID-19 has had on Public Health worldwide acknowledges the importance of pursuing multidisciplinary research efforts needed to understand the pathogen and control the disease.
Slightly over 2 years after the first cases of infection were reported in Wuhan, China, the new SARS-CoV-2 coronavirus, cause of an entirely new disease COVID-19, had infected over 500 million people, and directly resulted in the loss of 6 million human lives. The COVID-19 fatality rate exceeds that of malaria, HIV/AIDS, and influenza, and within the first year of the pandemic, the yearly fatality rate of rapidly approached that of the deadliest human infectious disease, TB. Thus, the SARS-CoV-2 pandemic has had an indirect negative impact on other areas of Public Health, causing a global setback in the 2030 Sustainable Development Goals, and although several vaccines have been approved for public use, it is apparent that the struggle to control the COVID-19 pandemic will not subside for some time to come.
Throughout human history, deadly infections have shaped our immune system. However, it is still naïve, at least partially, in relation to the new virus SARS-CoV-2. SARS-CoV-2 has gradually acquired several mutations that determined the emergence of new variants characterized by different phenotypes (e.g., with changes in transmissibility, pathogenicity and antigenicity or immune escape ability). To date, however, no specific mutations have been found in SARS-CoV-2 that could explain the extraordinary range in the clinical forms of COVID-19. It is therefore likely that, in human beings, a genetic basis could govern severe COVID-19. Nevertheless, it remains of utmost importance to routinely monitor SARS-CoV-2 mutations and investigate the relationship between viral genetic variants and the clinical features/ disease phenotype through sequence-based surveillance, epidemiological studies, and clinical and research laboratory investigations (CDC 2021 ; Bakhshandeh et al. 2021 ; Duarte et al. 2021 ; Zhou and Wang 2021 ).
Currently, the scientific community is making important efforts to elucidate the molecular mechanisms of the pathophysiology of SARS-CoV-2 infection and as more results from genome-wide approaches are available through the ongoing global collaborative efforts; these will undeniably identify target genes, genomic regions and molecular pathways that will contribute to the current understanding of the genetic determinants of COVID-19. A greater awareness of the importance of candidate genes identified by genome-wide datasets is viewed as an imperative for the future success of these efforts. In the current theory of human infection genetics, a homogeneous physiological basis in the molecular pathways that govern the genetics of serious forms of COVID-19 is to be expected. The identification of genes associated with disease severity can flag the main molecular pathways involved, providing an abundance of information from which to select candidate genes for future studies and therapeutic developments. However, the moderate effect sizes of associated common variants from genome-wide approaches make it difficult to establish a functional basis. Therefore, there is an increased need for replication strategies by which to infer causality. The strategic use of meta-analysis of genome-wide overlap, such as the MAIC, or other meta-analytical algorithms still need further development as tools for candidate gene selection and validation.
Except for the interferon circuit genes, there is currently insufficient data, to identify risk and/or resistance profiles for screening with practical implications on Public Health. The combined knowledge of these efforts is at the basis of scientific developments for the future control of COVID-19. Ultimately, such knowledge has the potential to prompt the identification of promising viral- or host-directed targets and to establish an integrated COVID-19 therapeutic discovery and development program (Stevens 2004 ; Hingorani et al. 2019 ; Seyhan 2019 ; Trigueiro-Louro et al. 2019 , 2020 ; Parkinson et al. 2020 ; CDC 2021 ).
Publisher's Note
Springer Nature remains neutral with regard to jurisdictional claims in published maps and institutional affiliations.
- Akira S, Uematsu S, Takeuchi O. Pathogen recognition and innate immunity. Cell. 2006; 124 (4):783–801. doi: 10.1016/j.cell.2006.02.015. [ PubMed ] [ CrossRef ] [ Google Scholar ]
- Alangari AA, Al-Zamil F, Al-Mazrou A et al (2011) Treatment of disseminated mycobacterial infection with high-dose IFN-γ in a patient with IL-12Rβ1 deficiency. Clin Dev Immunol 2011:691956. [ PMC free article ] [ PubMed ]
- Amati F, Vancheri C, Latini A, Colona VL, Grelli S, D'Apice MR et al (2020) Expression profiles of the SARS-CoV-2 host invasion genes in nasopharyngeal and oropharyngeal swabs of COVID-19 patients. Heliyon 6(10):e05143 [ PMC free article ] [ PubMed ]
- Anastassopoulou C, Gkizarioti Z, Patrinos GP, Tsakris A. Human genetic factors associated with susceptibility to SARS-CoV-2 infection and COVID-19 disease severity. Hum Genomics. 2020; 14 (1):1–8. doi: 10.1186/s40246-020-00290-4. [ PMC free article ] [ PubMed ] [ CrossRef ] [ Google Scholar ]
- Ansari MA, Marchi E, Ramamurthy N et al (2020) Negative regulation of ACE2 by interferons in vivo and its genetic control medRxiv. 10.1101/2020.04.26.20080408
- Asselta R, Paraboschi EM, Mantovani A, Duga S. ACE2 and TMPRSS2 variants and expression as candidates to sex and country differences in COVID-19 severity in Italy. Aging (albany NY) 2020; 12 (11):10087. doi: 10.18632/aging.103415. [ PMC free article ] [ PubMed ] [ CrossRef ] [ Google Scholar ]
- Aykac K, Cura Yayla BC, Ozsurekci Y, et al. The association of viral load and disease severity in children with COVID-19. J Med Virol. 2021; 93 (5):3077–3083. doi: 10.1002/jmv.26853. [ PMC free article ] [ PubMed ] [ CrossRef ] [ Google Scholar ]
- Bai Y, Yao L, Wei T, et al. Presumed asymptomatic carrier transmission of COVID-19. JAMA. 2020; 323 (14):1406–1407. doi: 10.1001/jama.2020.2565. [ PMC free article ] [ PubMed ] [ CrossRef ] [ Google Scholar ]
- Baillie JK. Targeting the host immune response to fight infection. Science. 2014; 344 :807–808. doi: 10.1126/science.1255074. [ PubMed ] [ CrossRef ] [ Google Scholar ]
- Baggen J, Persoons L, Vanstreels E, et al. Genome-wide CRISPR screening identifies TMEM106B as a proviral host factor for SARS-CoV-2. Nat Genet. 2021; 53 :435–444. doi: 10.1038/s41588-021-00805-2. [ PubMed ] [ CrossRef ] [ Google Scholar ]
- Bakhshandeh B, Jahanafrooz Z, Abbasi A et al (2021) Mutations in SARS-CoV-2; Consequences in structure function and pathogenicity of the virus. Microb Pathog 154:104831 [ PMC free article ] [ PubMed ]
- Barbeira AN, Dickinson SP, Bonazzola R, et al. Exploring the phenotypic consequences of tissue specific gene expression variation inferred from GWAS summary statistics. Nat Commun. 2018; 9 (1):1–20. doi: 10.1038/s41467-018-03621-1. [ PMC free article ] [ PubMed ] [ CrossRef ] [ Google Scholar ]
- Barreiro LB, Ben-Ali M, Quach H et al (2009) Evolutionary dynamics of human Toll-like receptors and their different contributions to host defense. PLoS Genet 5(7):e1000562 [ PMC free article ] [ PubMed ]
- Bastard P, Rosen LB, Zhang Q et al (2020) Autoantibodies against type I IFNs in patients with life-threatening COVID-19. Science 370(6515):eabd4585 [ PMC free article ] [ PubMed ]
- Belouzard S, Millet JK, Licitra BN, Whittaker GR. Mechanisms of coronavirus cell entry mediated by the viral spike protein. Viruses. 2012; 4 (6):1011–1033. doi: 10.3390/v4061011. [ PMC free article ] [ PubMed ] [ CrossRef ] [ Google Scholar ]
- Benetti E, Tita R, Spiga O, et al. ACE2 gene variants may underlie interindividual variability and susceptibility to COVID-19 in the Italian population. Eur J Hum Genet. 2020; 28 (11):1602–1614. doi: 10.1038/s41431-020-0691-z. [ PMC free article ] [ PubMed ] [ CrossRef ] [ Google Scholar ]
- Bojkova D, McGreig JE, et al. SARS-CoV-2 and SARS-CoV differ in their cell tropism and drug sensitivity profiles. BioRxiv. 2020 doi: 10.1101/2020.04.03.024257. [ CrossRef ] [ Google Scholar ]
- Butler-Laporte G, Nakanishi T, Mooser V, et al. The effect of angiotensin-converting enzyme levels on Covid-19 susceptibility and severity: a Mendelian randomization study. Int J Epidemiol. 2021; 50 (1):75–86. doi: 10.1093/ije/dyaa229. [ PMC free article ] [ PubMed ] [ CrossRef ] [ Google Scholar ]
- Burke RM, Midgley CM, Dratch A, et al. Active monitoring of persons exposed to patients with confirmed COVID-19—United States, January–February 2020. Morb Mortal Wkly Rep. 2020; 69 (9):245. doi: 10.15585/mmwr.mm6909e1. [ PMC free article ] [ PubMed ] [ CrossRef ] [ Google Scholar ]
- Cao Y, Li L, Feng Z, et al. Comparative genetic analysis of the novel coronavirus (2019-nCoV/SARS-CoV-2) receptor ACE2 in different populations. Cell Disc. 2020; 6 (1):1–4. [ PMC free article ] [ PubMed ] [ Google Scholar ]
- Carter-Timofte ME, Jørgensen SE, Freytag MR, et al. Deciphering the role of host genetics in susceptibility to severe COVID-19. Front Immunol. 2020; 11 :1606. doi: 10.3389/fimmu.2020.01606. [ PMC free article ] [ PubMed ] [ CrossRef ] [ Google Scholar ]
- Casanova JL, Conley ME, Seligman SJ, et al. Guidelines for genetic studies in single patients: lessons from primary immunodeficiencies. J Exp Med. 2014; 211 (11):2137–2149. doi: 10.1084/jem.20140520. [ PMC free article ] [ PubMed ] [ CrossRef ] [ Google Scholar ]
- Casanova JL, Abel L. The human genetic determinism of life-threatening infectious diseases: genetic heterogeneity and physiological homogeneity? Hum Genet. 2020; 139 :681–694. doi: 10.1007/s00439-020-02184-w. [ PMC free article ] [ PubMed ] [ CrossRef ] [ Google Scholar ]
- Casanova JL, Su HC, Abel L, et al. A global effort to define the human genetics of protective immunity to SARS-CoV-2 infection. Cell. 2020; 181 (6):1194–1199. doi: 10.1016/j.cell.2020.05.016. [ PMC free article ] [ PubMed ] [ CrossRef ] [ Google Scholar ]
- Casanova JL, Abel L. Lethal infectious diseases as inborn errors of immunity: toward a synthesis of the germ and genetic theories. Ann Rev Pathol. 2021; 16 :23–50. doi: 10.1146/annurev-pathol-031920-101429. [ PMC free article ] [ PubMed ] [ CrossRef ] [ Google Scholar ]
- Centers for Disease Control and Prevention, CDC (2021). SARS-CoV-2 variant classifications and definitions. https://www.cdc.gov/coronavirus/2019-ncov/variants/variant-info.html#Interest . Accessed 19 Nov 2021
- Ciancanelli MJ, Huang SX, Luthra P, et al. Life-threatening influenza and impaired interferon amplification in human IRF7 deficiency. Science. 2015; 348 (6233):448–453. doi: 10.1126/science.aaa1578. [ PMC free article ] [ PubMed ] [ CrossRef ] [ Google Scholar ]
- Chan RW, Chan MC, Agnihothram S, et al. Tropism of and innate immune responses to the novel human betacoronavirus lineage C virus in human ex vivo respiratory organ cultures. J Virol. 2013; 87 (12):6604–6614. doi: 10.1128/JVI.00009-13. [ PMC free article ] [ PubMed ] [ CrossRef ] [ Google Scholar ]
- Chan JF, Lau SK, To KK, Cheng VC, et al. Middle East respiratory syndrome coronavirus: another zoonotic betacoronavirus causing SARS-like disease. Clin Microbiol Rev. 2015; 28 :465–522. doi: 10.1128/CMR.00102-14. [ PMC free article ] [ PubMed ] [ CrossRef ] [ Google Scholar ]
- Channappanavar R, Perlman S. Pathogenic human coronavirus infections: causes and consequences of cytokine storm and immunopathology. Semin Immunopathol. 2017; 39 (5):529–539. doi: 10.1007/s00281-017-0629-x. [ PMC free article ] [ PubMed ] [ CrossRef ] [ Google Scholar ]
- Channappanavar R, Fehr AR, Zheng J, et al. IFN-I response timing relative to virus replication determines MERS coronavirus infection outcomes. J Clin Invest. 2019; 129 :3625–3639. doi: 10.1172/JCI126363. [ PMC free article ] [ PubMed ] [ CrossRef ] [ Google Scholar ]
- Chau NVV, Thanh Lam V, Thanh Dung N, et al. The natural history and transmission potential of asymptomatic SARS-CoV-2 infection. Clin Infect Dis. 2020; 71 (10):2679–2687. doi: 10.1093/cid/ciaa711. [ PMC free article ] [ PubMed ] [ CrossRef ] [ Google Scholar ]
- Chen X, Liu S, Goraya MU, et al. Host immune response to influenza A virus infection. Front Immunol. 2018; 9 :320. doi: 10.3389/fimmu.2018.00320. [ PMC free article ] [ PubMed ] [ CrossRef ] [ Google Scholar ]
- Chen Y, Liu Q, Guo D. Emerging coronaviruses: genome structure, replication, and pathogenesis. J Med Virol. 2020; 92 (4):418–423. doi: 10.1002/jmv.25681. [ PMC free article ] [ PubMed ] [ CrossRef ] [ Google Scholar ]
- Cheng Z, Zhou J, To KKW, et al. Identification of TMPRSS2 as a susceptibility gene for severe 2009 pandemic A (H1N1) influenza and A (H7N9) influenza. J Infect Dis. 2015; 212 (8):1214–1221. doi: 10.1093/infdis/jiv246. [ PMC free article ] [ PubMed ] [ CrossRef ] [ Google Scholar ]
- Cilloni L, Fu H, Vesga JF et al (2020) The potential impact of the COVID-19 pandemic on the tuberculosis epidemic a modelling analysis. E Clin Med 28:100603 [ PMC free article ] [ PubMed ]
- Cho NJ, Glenn JS. Materials science approaches in the development of broad-spectrum antiviral therapies. Nat Mater. 2020; 19 (8):813–816. doi: 10.1038/s41563-020-0698-4. [ PubMed ] [ CrossRef ] [ Google Scholar ]
- Chou J, Thomas PG, Randolph AG (2022) Immunology of SARS-CoV-2 infection in children. Nat Immunol 1–9 [ PMC free article ] [ PubMed ]
- Chu H, Zhou J, Wong BHY, et al. Middle East respiratory syndrome coronavirus efficiently infects human primary T lymphocytes and activates the extrinsic and intrinsic apoptosis pathways. J Infect Dis. 2016; 213 (6):904–914. doi: 10.1093/infdis/jiv380. [ PMC free article ] [ PubMed ] [ CrossRef ] [ Google Scholar ]
- Chwieralski CE, Welte T, Bühling F. Cathepsin-regulated apoptosis. Apoptosis. 2006; 11 (2):143–149. doi: 10.1007/s10495-006-3486-y. [ PubMed ] [ CrossRef ] [ Google Scholar ]
- Coutard B, Valle C, de Lamballerie X et al (2020) The spike glycoprotein of the new coronavirus 2019-nCoV contains a furin-like cleavage site absent in CoV of the same clade. Antivir Res 176:104742 [ PMC free article ] [ PubMed ]
- Colona VL, Vasiliou V, Watt J, Novelli G, Reichardt JK. Update on human genetic susceptibility to COVID-19: susceptibility to virus and response. Hum Genomics. 2021; 15 (1):1–8. [ PMC free article ] [ PubMed ] [ Google Scholar ]
- Colona VL, Biancolella M, Novelli A, Novelli G (2021b) Will GWAS eventually allow the identification of genomic biomarkers for COVID-19 severity and mortality? J Clin Invest 131(23) [ PMC free article ] [ PubMed ]
- COVID-19 Host Genetics Initiative (2021) Mapping the human genetic architecture of COVID-19 by worldwide meta-analysis MedRxiv. 10.1101/2021.03.10.21252820
- COVID-19 Host Genetics Initiative (2020) The COVID-19 host genetics initiative a global initiative to elucidate the role of host genetic factors in susceptibility and severity of the SARS-CoV-2 virus pandemic. Eur J Hum Genet 28(6):715 [ PMC free article ] [ PubMed ]
- Dahdouh E, Lázaro-Perona F, Romero-Gómez MP, et al. Ct values from SARS-CoV-2 diagnostic PCR assays should not be used as direct estimates of viral load. J Infect. 2021; 82 (3):414–451. doi: 10.1016/j.jinf.2020.10.017. [ PMC free article ] [ PubMed ] [ CrossRef ] [ Google Scholar ]
- Dandekar AA, Perlman S. Immunopathogenesis of coronavirus infections: implications for SARS. Nat Rev Immunol. 2005; 5 (12):917–927. doi: 10.1038/nri1732. [ PMC free article ] [ PubMed ] [ CrossRef ] [ Google Scholar ]
- Daniloski Z, Jordan TX, Wessels HH, et al. Identification of required host factors for SARS-CoV-2 infection in human cells. Cell. 2021; 184 (1):92–105. doi: 10.1016/j.cell.2020.10.030. [ PMC free article ] [ PubMed ] [ CrossRef ] [ Google Scholar ]
- David S. A current guide to candidate gene association studies. Trends Genet. 2021; 37 (12):1056–1059. doi: 10.1016/j.tig.2021.07.009. [ PubMed ] [ CrossRef ] [ Google Scholar ]
- David A, Parkinson N, Peacock TP, Pairo-Castineira E, Khanna T, Cobat A et al (2022) A common TMPRSS2 variant has a protective effect against severe COVID-19. Current research in translational medicine 70(2):103333 [ PMC free article ] [ PubMed ]
- Davies NG, Abbott S, Barnard RC et al (2020) Estimated transmissibility and severity of novel SARS-CoV-2 Variant of Concern 202012/01 in England. Science 372(6538) [ PMC free article ] [ PubMed ]
- Debnath M, Banerjee M, Berk M. Genetic gateways to COVID-19 infection: Implications for risk severity and outcomes. FASEB J. 2020; 34 (7):8787–8795. doi: 10.1096/fj.202001115R. [ PMC free article ] [ PubMed ] [ CrossRef ] [ Google Scholar ]
- De Castro MAG, Bunt G, Wouters FS. Cathepsin B launches an apoptotic exit effort upon cell death-associated disruption of lysosomes. Cell Death Discov. 2016; 2 (1):1–8. doi: 10.1038/cddiscovery.2016.12. [ PMC free article ] [ PubMed ] [ CrossRef ] [ Google Scholar ]
- De Wilde AH, Snijder EJ, Kikkert M, van Hemert MJ. Host factors in coronavirus replication. Curr Top Microbiol Immunol. 2018; 419 :1–42. [ PMC free article ] [ PubMed ] [ Google Scholar ]
- Devaux CA, Rolain JM, Raoult D. ACE2 receptor polymorphism: Susceptibility to SARS-CoV-2 hypertension multi-organ failure and COVID-19 disease outcome. J Microbiol Immunol Infect. 2020; 53 (3):425–435. doi: 10.1016/j.jmii.2020.04.015. [ PMC free article ] [ PubMed ] [ CrossRef ] [ Google Scholar ]
- Diao B, Wang C, Tan Y, et al. Reduction and functional exhaustion of T cells in patients with coronavirus disease 2019 (COVID-19) Front Immunol. 2020; 11 :827. doi: 10.3389/fimmu.2020.00827. [ PMC free article ] [ PubMed ] [ CrossRef ] [ Google Scholar ]
- Dong E, Du H, Gardner L. An interactive web-based dashboard to track COVID-19 in real time. Lancet Infect Dis. 2020; 20 (5):533–534. doi: 10.1016/S1473-3099(20)30120-1. [ PMC free article ] [ PubMed ] [ CrossRef ] [ Google Scholar ]
- Donnelly CA, Malik MR, Elkholy A, et al. Worldwide reduction in MERS cases and deaths since 2016. Emerg Infect Dis. 2019; 25 (9):1758. doi: 10.3201/eid2509.190143. [ PMC free article ] [ PubMed ] [ CrossRef ] [ Google Scholar ]
- Duarte CM, Jamil T, Gojobori T, Alam I (2021) Detection of SARS-CoV-2 variants requires urgent global coordination Int J Infect Dis 109:50–53 [ PMC free article ] [ PubMed ]
- Escobedo A, Kaushal D, Singh K (2022) Insights into the changing landscape of coronavirus disease 2019. Front Cell Infect Microbiol 11 [ PMC free article ] [ PubMed ]
- European Centre for Disease Prevention and Control, ECDC (2020) Detection of new SARS-CoV-2 variants related to mink. https://www.ecdc.europa.eu/sites/default/files/documents/RRA-SARS-CoV-2-in-mink-12-nov-2020.pdf
- Elhabyan A, El Yaacoub S, Sanad et al (2020) The role of host genetics in susceptibility to severe viral infections in humans and insights into host genetics of severe COVID-19: A systematic review. Virus Res 289:198163 [ PMC free article ] [ PubMed ]
- Ellinghaus D, Degenhardt F, Bujanda L, et al. Severe Covid-19 GWAS Group Genomewide association study of severe Covid-19 with respiratory failure. N Engl J Med. 2020; 383 (16):1522–1534. doi: 10.1056/NEJMoa2020283. [ PMC free article ] [ PubMed ] [ CrossRef ] [ Google Scholar ]
- European Centers for Disease Control and Prevention, ECDC (2022) SARS-CoV-2 variants of concern as of 17 February 2022. https://www.ecdc.europa.eu/en/covid-19/variants-concern . Accessed 26 Feb 2022
- Fajnzylber J, Regan J, Coxen K, et al. SARS-CoV-2 viral load is associated with increased disease severity and mortality. Nat Commun. 2020; 11 (1):1–9. doi: 10.1038/s41467-020-19057-5. [ PMC free article ] [ PubMed ] [ CrossRef ] [ Google Scholar ]
- Forni G, Mantovani A. COVID-19 vaccines: where we stand and challenges ahead. Cell Death Differ. 2021; 28 (2):626–639. doi: 10.1038/s41418-020-00720-9. [ PMC free article ] [ PubMed ] [ CrossRef ] [ Google Scholar ]
- Gadi N, Wu SC, Spihlman AP, Moulton VR. What’s sex got to do with COVID-19? Gender-based differences in the host immune response to coronaviruses. Front Immunol. 2020; 11 :2147. doi: 10.3389/fimmu.2020.02147. [ PMC free article ] [ PubMed ] [ CrossRef ] [ Google Scholar ]
- Ghinai I, Woods S, Ritger KA, et al. Community transmission of SARS-CoV-2 at two family gatherings—Chicago, Illinois, February–March 2020. Morb Mortal Wkly Rep. 2020; 69 (15):446. doi: 10.15585/mmwr.mm6915e1. [ PMC free article ] [ PubMed ] [ CrossRef ] [ Google Scholar ]
- Golinelli D, Boetto E, Maietti E, Fantini MP (2020) The association between ABO blood group and SARS-CoV-2 infection: A meta-analysis. PLoS One 15(9):e0239508 [ PMC free article ] [ PubMed ]
- Godri Pollitt KJ, Peccia J, Ko AI, et al. COVID-19 vulnerability: the potential impact of genetic susceptibility and airborne transmission. Hum Genomics. 2020; 14 :1–7. doi: 10.1186/s40246-020-00267-3. [ PMC free article ] [ PubMed ] [ CrossRef ] [ Google Scholar ]
- Gómez J, Albaiceta GM, Cuesta-Llavona E, García-Clemente M, López-Larrea C, Amado-Rodríguez L et al (2021) The Interferon-induced transmembrane protein 3 gene (IFITM3) rs12252 C variant is associated with COVID-19. Cytokine 137:155354 [ PubMed ]
- Grant WB, Lahore H, McDonnell SL, et al. Evidence that vitamin D supplementation could reduce risk of influenza and COVID-19 infections and deaths. Nutrients. 2020; 12 (4):988. doi: 10.3390/nu12040988. [ PMC free article ] [ PubMed ] [ CrossRef ] [ Google Scholar ]
- Hadjadj J, Yatim N, Barnabei L, et al. Impaired type I interferon activity and inflammatory responses in severe COVID-19 patients. Science. 2020; 369 (6504):718–724. doi: 10.1126/science.abc6027. [ PMC free article ] [ PubMed ] [ CrossRef ] [ Google Scholar ]
- Hakonarson H, Gulcher JR, Stefansson K (2003) deCODE genetics Inc. Pharmacogenomics 4(2):209–215 [ PubMed ]
- Hamner L (2020) High SARS-CoV-2 attack rate following exposure at a choir practice-Skagit County, Washington, March 2020. MMWR Morbidity and mortality weekly report 69 [ PubMed ]
- Hasanoglu I, Korukluoglu G, Asilturk D, et al. Higher viral loads in asymptomatic COVID-19 patients might be the invisible part of the iceberg. Infection. 2021; 49 (1):117–126. doi: 10.1007/s15010-020-01548-8. [ PMC free article ] [ PubMed ] [ CrossRef ] [ Google Scholar ]
- He X, Hong W, Pan X, et al. SARS-CoV-2 Omicron variant: characteristics and prevention. MedComm. 2021; 2 :838–845. doi: 10.1002/mco2.110. [ PMC free article ] [ PubMed ] [ CrossRef ] [ Google Scholar ]
- He R, Lu Z, Zhang L et al (2020) The clinical course and its correlated immune status in COVID-19 pneumonia. J Clin Virol 127:104361 [ PMC free article ] [ PubMed ]
- Hebbring SJ. The challenges, advantages and future of phenome-wide association studies. Immunology. 2014; 141 (2):157–165. doi: 10.1111/imm.12195. [ PMC free article ] [ PubMed ] [ CrossRef ] [ Google Scholar ]
- Heald-Sargent T, Gallagher T. Ready, set, fuse! The coronavirus spike protein and acquisition of fusion competence. Viruses. 2012; 4 (4):557–580. doi: 10.3390/v4040557. [ PMC free article ] [ PubMed ] [ CrossRef ] [ Google Scholar ]
- Hingorani AD, Kuan V, Finan C, et al. Improving the odds of drug development success through human genomics: modelling study. Sci Rep. 2019; 9 (1):1–25. doi: 10.1038/s41598-019-54849-w. [ PMC free article ] [ PubMed ] [ CrossRef ] [ Google Scholar ]
- Hoehl S, Rabenau H, Berger A, et al. Evidence of SARS-CoV-2 infection in returning travelers from Wuhan. China N Engl J Med. 2020; 382 (13):1278–1280. doi: 10.1056/NEJMc2001899. [ PMC free article ] [ PubMed ] [ CrossRef ] [ Google Scholar ]
- Hofmann H, Pyrc K, Van Der Hoek L, et al. Human coronavirus NL63 employs the severe acute respiratory syndrome coronavirus receptor for cellular entry. Proc Natl Acad Sci U S A. 2005; 102 (22):7988–7993. doi: 10.1073/pnas.0409465102. [ PMC free article ] [ PubMed ] [ CrossRef ] [ Google Scholar ]
- Hoffmann M, Kleine-Weber H, Schroeder S, et al. SARS-CoV-2 cell entry depends on ACE2 and TMPRSS2 and is blocked by a clinically proven protease inhibitor. Cell. 2020; 181 (2):271–280. doi: 10.1016/j.cell.2020.02.052. [ PMC free article ] [ PubMed ] [ CrossRef ] [ Google Scholar ]
- Holland SM. Immunotherapy of mycobacterial infections. Semin Respir Infect. 2001; 16 (1):47–59. doi: 10.1053/srin.2001.22728. [ PubMed ] [ CrossRef ] [ Google Scholar ]
- Hussain M, Jabeen N, Raza F, et al. Structural variations in human ACE2 may influence its binding with SARS-CoV-2 spike protein. J Med Virol. 2020; 92 (9):1580–1586. doi: 10.1002/jmv.25832. [ PMC free article ] [ PubMed ] [ CrossRef ] [ Google Scholar ]
- Huang C, Wang Y, Li X, et al. Clinical features of patients infected with 2019 novel coronavirus in Wuhan. China Lancet. 2020; 395 (10223):497–506. doi: 10.1016/S0140-6736(20)30183-5. [ PMC free article ] [ PubMed ] [ CrossRef ] [ Google Scholar ]
- Hui KP, Cheung MC, Perera RA, et al. Tropism, replication competence, and innate immune responses of the coronavirus SARS-CoV-2 in human respiratory tract and conjunctiva: an analysis in ex-vivo and in-vitro cultures. Lancet Resp Med. 2020; 8 (7):687–695. doi: 10.1016/S2213-2600(20)30193-4. [ PMC free article ] [ PubMed ] [ CrossRef ] [ Google Scholar ]
- Jewell BL, Mudimu E, Stover J, et al. Potential effects of disruption to HIV programmes in sub-Saharan Africa caused by COVID-19: results from multiple mathematical models. Lancet HIV. 2020; 7 (9):e629–e640. doi: 10.1016/S2352-3018(20)30211-3. [ PMC free article ] [ PubMed ] [ CrossRef ] [ Google Scholar ]
- Jiang L, Tang K, Levin M, et al. COVID-19 and multisystem inflammatory syndrome in children and adolescents. Lancet Infect Dis. 2020; 20 (11):e276–e288. doi: 10.1016/S1473-3099(20)30651-4. [ PMC free article ] [ PubMed ] [ CrossRef ] [ Google Scholar ]
- Kanehisa M, Goto S. KEGG: kyoto encyclopedia of genes and genomes. Nucleic Acids Res. 2000; 28 (1):27–30. doi: 10.1093/nar/28.1.27. [ PMC free article ] [ PubMed ] [ CrossRef ] [ Google Scholar ]
- Karim SSA, Karim QA. Omicron SARS-CoV-2 variant: a new chapter in the COVID-19 pandemic. Lancet. 2021; 398 (10317):2126–2128. doi: 10.1016/S0140-6736(21)02758-6. [ PMC free article ] [ PubMed ] [ CrossRef ] [ Google Scholar ]
- Kato H, Sato S, Yoneyama M, et al. Cell type-specific involvement of RIG-I in antiviral response. Immunity. 2005; 23 (1):19–28. doi: 10.1016/j.immuni.2005.04.010. [ PubMed ] [ CrossRef ] [ Google Scholar ]
- Kawai T, Akira S. Toll-like receptor and RIG-1-like receptor signaling. Ann N Y Acad Sci. 2008; 1143 :1–20. doi: 10.1196/annals.1443.020. [ PubMed ] [ CrossRef ] [ Google Scholar ]
- King EA, Davis JW, Degner JF (2019) Are drug targets with genetic support twice as likely to be approved? Revised estimates of the impact of genetic support for drug mechanisms on the probability of drug approval. PLoS Genet 15(12):e1008489 [ PMC free article ] [ PubMed ]
- Kielian M, Rey FA. Virus membrane-fusion proteins: more than one way to make a hairpin. Nature Rev Microbiol. 2006; 4 (1):67–76. doi: 10.1038/nrmicro1326. [ PMC free article ] [ PubMed ] [ CrossRef ] [ Google Scholar ]
- Khateeb J, Li Y, Zhang H. Emerging SARS-CoV-2 variants of concern and potential intervention approaches. Crit Care. 2021; 25 (1):1–8. doi: 10.1186/s13054-021-03662-x. [ PMC free article ] [ PubMed ] [ CrossRef ] [ Google Scholar ]
- Koh WC, Naing L, Chaw L et al (2020) What do we know about SARS-CoV-2 transmission? A systematic review and meta-analysis of the secondary attack rate and associated risk factors. PloS one 15(10):e0240205 [ PMC free article ] [ PubMed ]
- Kousathanas A, Pairo-Castineira E, Rawlik K, Stuckey A, Odhams CA, Walker S et al (2022) Whole genome sequencing reveals host factors underlying critical Covid-19. Nature 1–10 [ PMC free article ] [ PubMed ]
- Kuo CL, Pilling LC, Atkins JL, et al. APOE e4 genotype predicts severe COVID-19 in the UK Biobank community cohort. J Gerontol A Biol Sci Med Sci. 2020; 75 (11):2231–2232. doi: 10.1093/gerona/glaa131. [ PMC free article ] [ PubMed ] [ CrossRef ] [ Google Scholar ]
- Kuo Lili, Godeke Gert-Jan, Raamsman Martin J. B., Masters Paul S., Rottier Peter J. M. Retargeting of Coronavirus by Substitution of the Spike Glycoprotein Ectodomain: Crossing the Host Cell Species Barrier. Journal of Virology. 2000; 74 (3):1393–1406. doi: 10.1128/JVI.74.3.1393-1406.2000. [ PMC free article ] [ PubMed ] [ CrossRef ] [ Google Scholar ]
- Latini A, Agolini E, Novelli A, Borgiani P, Giannini R, Gravina P et al (2020) COVID-19 and genetic variants of protein involved in the SARS-CoV-2 entry into the host cells. Genes 11(9):1010 [ PMC free article ] [ PubMed ]
- Lauring AS, Hodcroft EB. Genetic variants of SARS-CoV-2—what do they mean? JAMA. 2021; 325 (6):529–531. doi: 10.1001/jama.2020.27124. [ PubMed ] [ CrossRef ] [ Google Scholar ]
- Lavillegrand JR, Garnier M, Spaeth A, et al. Elevated plasma IL-6 and CRP levels are associated with adverse clinical outcomes and death in critically ill SARS-CoV-2 patients: inflammatory response of SARS-CoV-2 patients. Ann Intensive Care. 2021; 11 (1):1–10. [ PMC free article ] [ PubMed ] [ Google Scholar ]
- Lee HJ (2021) An interactome landscape of SARS-CoV-2 virus-human protein-protein interactions by protein sequence-based multi-label classifiers. bioRxiv
- Leopardi S, Holmes EC, Gastaldelli M, et al. Interplay between co-divergence and cross-species transmission in the evolutionary history of bat coronaviruses. Infect Genet Evol. 2018; 58 :279–289. doi: 10.1016/j.meegid.2018.01.012. [ PMC free article ] [ PubMed ] [ CrossRef ] [ Google Scholar ]
- Li F. Structure function and evolution of coronavirus spike proteins. Annu Rev Virol. 2016; 3 :237–261. doi: 10.1146/annurev-virology-110615-042301. [ PMC free article ] [ PubMed ] [ CrossRef ] [ Google Scholar ]
- Li X, Geng M, Peng Y, et al. Molecular immune pathogenesis and diagnosis of COVID-19. J Pharm Anal. 2020; 10 (2):102–108. doi: 10.1016/j.jpha.2020.03.001. [ PMC free article ] [ PubMed ] [ CrossRef ] [ Google Scholar ]
- Li Q, Cao Z, Rahman P (2020b) Genetic variability of human angiotensin‐converting enzyme 2 (hACE2) among various ethnic populations. Mol Genet Genomic Med 8(8):e1344 [ PMC free article ] [ PubMed ]
- Li B, Clohisey SM, Chia BS, et al. Genome-wide CRISPR screen identifies host dependency factors for influenza A virus infection. Nat Commun. 2020; 11 (1):1–18. [ PMC free article ] [ PubMed ] [ Google Scholar ]
- Li Z, Jiang J, Ruan X, Tong Y, Xu S, Han L, Jianguo XuJ. The zoonotic and natural foci characteristics of SARS-CoV-2. Journal of Biosafety and Biosecurity. 2021; 3 (1):51–55. doi: 10.1016/j.jobb.2021.06.002. [ PMC free article ] [ PubMed ] [ CrossRef ] [ Google Scholar ]
- Liao QJ, Ye LB, Timani KA, et al. Activation of NF-κB by the full-length nucleocapsid protein of the SARS coronavirus. Acta Biochim Biophys Sin. 2005; 37 (9):607–612. doi: 10.1111/j.1745-7270.2005.00082.x. [ PMC free article ] [ PubMed ] [ CrossRef ] [ Google Scholar ]
- Liu J, Li S, Liu J et al (2020) Longitudinal characteristics of lymphocyte responses and cytokine profiles in the peripheral blood of SARS-CoV-2 infected patients. EBioMedicine 55:102763 [ PMC free article ] [ PubMed ]
- Lokugamage KG, Hage A, Schindewolf C, et al. Type I interferon susceptibility distinguishes SARS-CoV-2 from SARS-CoV. J Virol. 2020; 94 :e01410–e1420. doi: 10.1128/JVI.01410-20. [ PMC free article ] [ PubMed ] [ CrossRef ] [ Google Scholar ]
- Lonsdale J, Thomas J, Salvatore M, et al. The genotype-tissue expression (GTEx) project. Nat Genet. 2013; 45 (6):580–585. doi: 10.1038/ng.2653. [ PMC free article ] [ PubMed ] [ CrossRef ] [ Google Scholar ]
- Lopez Bernal J, Andrews N, Gower C, et al. Effectiveness of Covid-19 vaccines against the B 1617 2 (Delta) variant. N Engl J Med. 2021; 385 :585–594. doi: 10.1056/NEJMoa2108891. [ PMC free article ] [ PubMed ] [ CrossRef ] [ Google Scholar ]
- LoPresti M, Beck DB, Duggal P, et al. The role of host genetic factors in coronavirus susceptibility: review of animal and systematic review of human literature. Am J Hum Genet. 2020; 107 (3):381–402. doi: 10.1016/j.ajhg.2020.08.007. [ PMC free article ] [ PubMed ] [ CrossRef ] [ Google Scholar ]
- Luo CH, Morris CP, Sachithanandham J et al (2021) Infection with the SARS-CoV-2 delta variant is associated with higher infectious virus loads compared to the alpha variant in both unvaccinated and vaccinated individuals. medRxiv. 10.1101/2021.08.15.21262077
- Luliano AD, Roguski KM, Chang HH, et al. Estimates of global seasonal influenza-associated respiratory mortality: a modelling study. Lancet. 2018; 391 (10127):1285–1300. doi: 10.1016/S0140-6736(17)33293-2. [ PMC free article ] [ PubMed ] [ CrossRef ] [ Google Scholar ]
- Lu C, Gam R, Pandurangan AP, Gough J. Genetic risk factors for death with SARS-CoV-2 from the UK Biobank. MedRxiv. 2020 doi: 10.1101/2020.07.01.20144592. [ CrossRef ] [ Google Scholar ]
- Mahallawi WH, Khabour OF, Zhang Q, et al. MERS-CoV infection in humans is associated with a pro-inflammatory Th1 and Th17 cytokine profile. Cytokine. 2018; 104 :8–13. doi: 10.1016/j.cyto.2018.01.025. [ PMC free article ] [ PubMed ] [ CrossRef ] [ Google Scholar ]
- Marks M, Millat-Martinez P, Ouchi D, et al. Transmission of COVID-19 in 282 clusters in Catalonia Spain: a cohort study. Lancet Infect Dis. 2021; 21 :629–636. doi: 10.1016/S1473-3099(20)30985-3. [ PMC free article ] [ PubMed ] [ CrossRef ] [ Google Scholar ]
- McIntosh K, Hirsch MS, Bloom A (2022) Coronavirus disease 2019 (COVID-19): Epidemiology, virology, and prevention. Lancet Infect Dis 1:2019–20. https://www.uptodate.com/contents/covid-19-epidemiology-virology-and-prevention . Accessed 26 Feb 2022
- Meng T, Cao H, Zhang H, et al. The insert sequence in SARS-CoV-2 enhances spike protein cleavage by TMPRSS. BioRxiv. 2020 doi: 10.1101/2020.02.08.926006. [ CrossRef ] [ Google Scholar ]
- Merad M, Martin JC. Pathological inflammation in patients with COVID-19: a key role for monocytes and macrophages. Nat Rev Immunol. 2020; 20 (6):355–362. doi: 10.1038/s41577-020-0331-4. [ PMC free article ] [ PubMed ] [ CrossRef ] [ Google Scholar ]
- Millet JK, Whittaker GR. Host cell entry of Middle East respiratory syndrome coronavirus after two-step, furin-mediated activation of the spike protein. Proc Natl Acad Sci U S A. 2014; 111 :15214–15219. doi: 10.1073/pnas.1407087111. [ PMC free article ] [ PubMed ] [ CrossRef ] [ Google Scholar ]
- Minarowska A, Minarowski L, Karwowska A, Gacko M. Regulatory role of cathepsin D in apoptosis. Folia Histochem Cytobiol. 2007; 45 (3):159–163. [ PubMed ] [ Google Scholar ]
- Moon CY, Schilder, BM, Raj T, Huang KL (2021) Phenome-wide and expression quantitative trait locus associations of coronavirus disease 2019 genetic risk loci. Iscience 24(6):102550 [ PMC free article ] [ PubMed ]
- Nica AC, Dermitzakis ET. Expression quantitative trait loci: present and future. Philos Trans R Soc Lond B Biol Sci. 2013; 368 (1620):20120362. doi: 10.1098/rstb.2012.0362. [ PMC free article ] [ PubMed ] [ CrossRef ] [ Google Scholar ]
- Nicoli F, Solis-Soto MT, Paudel D, et al. Age-related decline of de novo T cell responsiveness as a cause of COVID-19 severity. Geroscience. 2020; 42 (4):1015–1019. doi: 10.1007/s11357-020-00217-w. [ PMC free article ] [ PubMed ] [ CrossRef ] [ Google Scholar ]
- Nguyen A, David JK, Maden SK, et al. Human leukocyte antigen susceptibility map for severe acute respiratory syndrome coronavirus 2. J Virol. 2020; 94 (13):e00510–e520. doi: 10.1128/JVI.00510-20. [ PMC free article ] [ PubMed ] [ CrossRef ] [ Google Scholar ]
- Novelli G, Liu J, Biancolella M, Alonzi T, Novelli A, Patten JJ, et al. Inhibition of HECT E3 ligases as potential therapy for COVID-19. Cell Death Dis. 2021; 12 (4):1–18. doi: 10.1038/s41419-021-03513-1. [ PMC free article ] [ PubMed ] [ CrossRef ] [ Google Scholar ]
- NSW (2021) Government. Living Evidence - SARS-CoV-2 variants. [WWW Document]. https://aci.health.nsw.gov.au/covid-19/critical-intelligence-unit/sars-cov-2-variants . Accessed 24 Jun 2021
- Oladejo BO, Adeboboye CF, Adebolu TT. Understanding the genetic determinant of severity in viral diseases: a case of SARS-Cov-2 infection. Egypt J Med Hum Genet. 2020; 21 (1):1–11. doi: 10.1186/s43042-020-00122-z. [ CrossRef ] [ Google Scholar ]
- Ortiz-Prado E, Simbaña-Rivera K, Gómez-Barreno L et al (2020) Clinical, molecular and epidemiological characterization of the SARS-CoV-2 virus and the Coronavirus Disease 2019 (COVID-19), a comprehensive literature review. Diagn Microbiol Infect Dis 98(1):115094 [ PMC free article ] [ PubMed ]
- Osuchowski MF, Winkler MS, Skirecki T, et al. The COVID-19 puzzle: deciphering pathophysiology and phenotypes of a new disease entity. Lancet Resp Med. 2021; 9 :622–642. doi: 10.1016/S2213-2600(21)00218-6. [ PMC free article ] [ PubMed ] [ CrossRef ] [ Google Scholar ]
- Ovsyannikova IG, Haralambieva IH, Crooke SN, et al. The role of host genetics in the immune response to SARS-CoV-2 and COVID-19 susceptibility and severity. Immunol Rev. 2020; 296 (1):205–219. doi: 10.1111/imr.12897. [ PMC free article ] [ PubMed ] [ CrossRef ] [ Google Scholar ]
- Pairo-Castineira E, Clohisey S, Klaric L, et al. Genetic mechanisms of critical illness in Covid-19. Nature. 2021; 591 (7848):92–98. doi: 10.1038/s41586-020-03065-y. [ PubMed ] [ CrossRef ] [ Google Scholar ]
- Pan D, Sze S, Minhas JS et al (2020) The impact of ethnicity on clinical outcomes in COVID-19: a systematic review. E Clin Med 23:100404 [ PMC free article ] [ PubMed ]
- Park SE. Epidemiology, virology, and clinical features of severe acute respiratory syndrome-coronavirus-2 (SARS-CoV-2; Coronavirus Disease-19) Clin Exp Pediatr. 2020; 63 (4):119. doi: 10.3345/cep.2020.00493. [ PMC free article ] [ PubMed ] [ CrossRef ] [ Google Scholar ]
- Parkinson N, Rodgers N, Fourman MH, et al. Systematic review and meta-analysis identifies potential host therapeutic targets in COVID-19. Sci Rep. 2020; 10 :22303. doi: 10.1038/s41598-020-79033-3. [ PMC free article ] [ PubMed ] [ CrossRef ] [ Google Scholar ]
- Peckham H, de Gruijter NM, Raine C, et al. Male sex identified by global COVID-19 meta-analysis as a risk factor for death and ITU admission. Nat Commun. 2020; 11 (1):1–10. doi: 10.1038/s41467-020-19741-6. [ PMC free article ] [ PubMed ] [ CrossRef ] [ Google Scholar ]
- Peng Y, Mentzer AJ, Liu G, et al. Broad and strong memory CD4+ and CD8+ T cells induced by SARS-CoV-2 in UK convalescent individuals following COVID-19. Nat Immunol. 2020; 21 (11):1336–1345. doi: 10.1038/s41590-020-0782-6. [ PMC free article ] [ PubMed ] [ CrossRef ] [ Google Scholar ]
- Phan MV, Ngo Tri T, Hong Anh P et al (2018) Identification and characterization of Coronaviridae genomes from Vietnamese bats and rats based on conserved protein domains. Virus Evol 4(2) vey035 [ PMC free article ] [ PubMed ]
- Platanias LC. Mechanisms of type-I-and type-II-interferon-mediated signaling. Nat Rev Immunol. 2005; 5 (5):375–386. doi: 10.1038/nri1604. [ PubMed ] [ CrossRef ] [ Google Scholar ]
- Pujadas E, Chaudhry F, McBride R et al (2020) SARS-CoV-2 viral load predicts COVID-19 mortality. Lancet Resp Med 8(9):e70 [ PMC free article ] [ PubMed ]
- Qin C, Zhou L, Hu Z, et al. Dysregulation of immune response in patients with COVID-19 in Wuhan. China Clin Infect Dis. 2020; 71 (15):762–768. doi: 10.1093/cid/ciaa248. [ PMC free article ] [ PubMed ] [ CrossRef ] [ Google Scholar ]
- Rausell A, Juan D, Pazos F, Valencia A. Protein interactions and ligand binding: from protein subfamilies to functional specificity. Proc Natl Acad Sci U S A. 2010; 107 (5):1995–2000. doi: 10.1073/pnas.0908044107. [ PMC free article ] [ PubMed ] [ CrossRef ] [ Google Scholar ]
- Repnik U, Stoka V, Turk V. Turk B (2012) Lysosomes and lysosomal cathepsins in cell death. Biochim Biophys Acta Proteins Proteom. 1824; 1 :22–33. [ PubMed ] [ Google Scholar ]
- Russo R, Andolfo I, Lasorsa VA, Cantalupo S, Marra R, Frisso G, et al. The TNFRSF13C H159Y variant is associated with severe COVID-19: a retrospective study of 500 patients from Southern Italy. Genes. 2021; 12 (6):881. doi: 10.3390/genes12060881. [ PMC free article ] [ PubMed ] [ CrossRef ] [ Google Scholar ]
- Sadler AJ, Williams BR. Interferon-inducible antiviral effectors. Nat Rev Immunol. 2008; 8 (7):559–568. doi: 10.1038/nri2314. [ PMC free article ] [ PubMed ] [ CrossRef ] [ Google Scholar ]
- Schoggins JW, Wilson SJ, Panis M, et al. A diverse range of gene products are effectors of the type I interferon antiviral response. Nature. 2011; 472 :481–485. doi: 10.1038/nature09907. [ PMC free article ] [ PubMed ] [ CrossRef ] [ Google Scholar ]
- SeyedAlinaghi S, Mehrtak M, MohsseniPour M, Mirzapour P, Barzegary A, Habibi P, et al. Genetic susceptibility of COVID-19: a systematic review of current evidence. Eur J Med Res. 2021; 26 (1):1–12. doi: 10.1186/s40001-021-00516-8. [ PMC free article ] [ PubMed ] [ CrossRef ] [ Google Scholar ]
- Shang J, Ye G, Shi K, et al. Structural basis of receptor recognition by SARS-CoV-2. Nature. 2020; 581 (7807):221–224. doi: 10.1038/s41586-020-2179-y. [ PMC free article ] [ PubMed ] [ CrossRef ] [ Google Scholar ]
- Shang J, Wan Y, Luo C, et al. Cell entry mechanisms of SARS-CoV-2. Proc Natl Acad Sci U S A. 2020; 117 :11727–11734. doi: 10.1073/pnas.2003138117. [ PMC free article ] [ PubMed ] [ CrossRef ] [ Google Scholar ]
- Shenoy S. SARS-CoV-2 (COVID-19), viral load and clinical outcomes; lessons learned one year into the pandemic: A systematic review. World J Crit Care Med. 2021; 10 (4):132. doi: 10.5492/wjccm.v10.i4.132. [ PMC free article ] [ PubMed ] [ CrossRef ] [ Google Scholar ]
- Simmons G, Gosalia DN, Rennekamp AJ, et al. Inhibitors of cathepsin L prevent severe acute respiratory syndrome coronavirus entry. Proc Natl Acad Sci U S A. 2005; 102 :11876–11881. doi: 10.1073/pnas.0505577102. [ PMC free article ] [ PubMed ] [ CrossRef ] [ Google Scholar ]
- Skol AD, Scott LJ, Abecasis GR, Boehnke M. Joint analysis is more efficient than replication-based analysis for two-stage genome-wide association studies. Nat Genet. 2006; 38 (2):209–213. doi: 10.1038/ng1706. [ PubMed ] [ CrossRef ] [ Google Scholar ]
- Sekine T, Perez-Potti A, Rivera-Ballesteros O, et al. Robust T cell immunity in convalescent individuals with asymptomatic or mild COVID-19. Cell. 2020; 183 (1):158–168. doi: 10.1016/j.cell.2020.08.017. [ PMC free article ] [ PubMed ] [ CrossRef ] [ Google Scholar ]
- Suh S, Lee S, Gym H, Yoon S, Park S, Cha J, et al. A systematic review on papers that study on Single Nucleotide Polymorphism that affects coronavirus 2019 severity. BMC Infect Dis. 2022; 22 (1):1–11. doi: 10.1186/s12879-022-07034-w. [ PMC free article ] [ PubMed ] [ CrossRef ] [ Google Scholar ]
- Stop TB Partnership (2020) The potential impact of the covid-19 response on tuberculosis in high-burden countries, a modelling analysis. https://www.stoptb.org/assets/documents/news/Modeling%20Report_1%20May%202020_FINAL.pdf
- Sun J, Zhuang Z, Zheng J, et al. Generation of a broadly useful model for COVID-19 pathogenesis vaccination and treatment. Cell. 2020; 182 (3):734–743. doi: 10.1016/j.cell.2020.06.010. [ PMC free article ] [ PubMed ] [ CrossRef ] [ Google Scholar ]
- Suri JS, Agarwal S, Gupta SK et al (2021) A narrative review on characterization of acute respiratory distress syndrome in COVID-19-infected lungs using artificial intelligence. Comput Biol Med 130:104210 [ PMC free article ] [ PubMed ]
- Seyhan AA. Lost in translation: the valley of death across preclinical and clinical divide–identification of problems and overcoming obstacles. Transl Med Commun. 2019; 4 (1):1–19. doi: 10.1186/s41231-019-0050-7. [ CrossRef ] [ Google Scholar ]
- Schneider WM, Luna JM, Hoffmann HH, et al. Genome-scale identification of SARS-CoV-2 and pan-coronavirus host factor networks. Cell. 2021; 184 (1):120–132. doi: 10.1016/j.cell.2020.12.006. [ PMC free article ] [ PubMed ] [ CrossRef ] [ Google Scholar ]
- Schrader K, Huai J, Jöckel L, et al. Non-caspase proteases: triggers or amplifiers of apoptosis? Cell Mol Life Sci. 2010; 67 (10):1607–1618. doi: 10.1007/s00018-010-0287-9. [ PubMed ] [ CrossRef ] [ Google Scholar ]
- Stoeger T, Amaral LAN (2020) Meta-Research: COVID-19 research risks ignoring important host genes due to pre-established research patterns. Elife 9:e61981 [ PMC free article ] [ PubMed ]
- Suryamohan K, Diwanji D, Stawiski EW, et al. Human ACE2 receptor polymorphisms and altered susceptibility to SARS-CoV-2. Commun Biol. 2021; 4 :475. doi: 10.1038/s42003-021-02030-3. [ PMC free article ] [ PubMed ] [ CrossRef ] [ Google Scholar ]
- Stevens RC. Long live structural biology. Nat Struct Mol Biol. 2004; 11 (4):293–295. doi: 10.1038/nsmb0404-293. [ PMC free article ] [ PubMed ] [ CrossRef ] [ Google Scholar ]
- Tang D, Kang R, Berghe TV, et al. The molecular machinery of regulated cell death. Cell Res. 2019; 29 (5):347–364. doi: 10.1038/s41422-019-0164-5. [ PMC free article ] [ PubMed ] [ CrossRef ] [ Google Scholar ]
- Tang D, Comish P, Kang R (2020) The hallmarks of COVID-19 disease. PLoS Pathog 16(5):e1008536 [ PMC free article ] [ PubMed ]
- Torre-Fuentes L, Matías-Guiu J, Hernández-Lorenzo L, Montero-Escribano P, Pytel V, Porta-Etessam J, et al. ACE2 TMPRSS2 and Furin variants and SARS-CoV-2 infection in Madrid Spain. J Med Virol. 2021; 93 (2):863–869. doi: 10.1002/jmv.26319. [ PMC free article ] [ PubMed ] [ CrossRef ] [ Google Scholar ]
- Trigueiro-Louro JM, Correia V, Santos LA, et al. To hit or not to hit: large-scale sequence analysis and structure characterization of influenza A NS1 unlocks new antiviral target potential. Virology. 2019; 535 :297–307. doi: 10.1016/j.virol.2019.04.009. [ PubMed ] [ CrossRef ] [ Google Scholar ]
- Trigueiro-Louro J, Correia V, Figueiredo-Nunes I, et al. Unlocking COVID therapeutic targets: A structure-based rationale against SARS-CoV-2, SARS-CoV and MERS-CoV Spike. Comput Struct Biotechnol J. 2020; 18 :2117–2131. doi: 10.1016/j.csbj.2020.07.017. [ PMC free article ] [ PubMed ] [ CrossRef ] [ Google Scholar ]
- Vabret N, Britton GJ, Gruber C, et al. Immunology of COVID-19: current state of the science. Immunity. 2020; 52 (6):910–941. doi: 10.1016/j.immuni.2020.05.002. [ PMC free article ] [ PubMed ] [ CrossRef ] [ Google Scholar ]
- Van Der Made CI, Simons A, Schuurs-Hoeijmakers J, et al. Presence of genetic variants among young men with severe COVID-19. JAMA. 2020; 324 (7):663–673. doi: 10.1001/jama.2020.13719. [ PMC free article ] [ PubMed ] [ CrossRef ] [ Google Scholar ]
- Walsh KA, Jordan K, Clyne B, et al. SARS-CoV-2 detection, viral load and infectivity over the course of an infection: SARS-CoV-2 detection, viral load and infectivity. J Infect. 2020; 81 (3):357–371. doi: 10.1016/j.jinf.2020.06.067. [ PMC free article ] [ PubMed ] [ CrossRef ] [ Google Scholar ]
- Walls AC, Park YJ, Tortorici MA, et al. Structure function and antigenicity of the SARS-CoV-2 spike glycoprotein. Cell. 2020; 181 (2):281–292. doi: 10.1016/j.cell.2020.02.058. [ PMC free article ] [ PubMed ] [ CrossRef ] [ Google Scholar ]
- Wang H, Ma S. The cytokine storm and factors determining the sequence and severity of organ dysfunction in multiple organ dysfunction syndrome. Am J Emerg Med. 2008; 26 (6):711–715. doi: 10.1016/j.ajem.2007.10.031. [ PubMed ] [ CrossRef ] [ Google Scholar ]
- Wang R, Simoneau CR, Kulsuptrakul J, et al. Genetic screens identify host factors for SARS-CoV-2 and common cold coronaviruses. Cell. 2021; 184 (1):106–119. doi: 10.1016/j.cell.2020.12.004. [ PMC free article ] [ PubMed ] [ CrossRef ] [ Google Scholar ]
- Weiner 3rd J, Suwalski P, Holtgrewe M, Rakitko A, Thibeault C, Müller M, Heidecker B (2021) Increased risk of severe clinical course of COVID-19 in carriers of HLA-C* 04: 01. EClinicalMedicine 40:101099 [ PMC free article ] [ PubMed ]
- Wei J, Alfajaro MM, DeWeirdt PC, et al. Genome-wide CRISPR screens reveal host factors critical for SARS-CoV-2 infection. Cell. 2021; 184 (1):76–91. doi: 10.1016/j.cell.2020.10.028. [ PMC free article ] [ PubMed ] [ CrossRef ] [ Google Scholar ]
- Weiskopf D, Schmitz KS, Raadsen MP et al (2020) Phenotype and kinetics of SARS-CoV-2–specific T cells in COVID-19 patients with acute respiratory distress syndrome. Sci Immunol 5(48):eabd2071 [ PMC free article ] [ PubMed ]
- Wong CK, Lam CWK, Wu AKL, et al. Plasma inflammatory cytokines and chemokines in severe acute respiratory syndrome. Clin Exp Immunol. 2004; 136 (1):95–103. doi: 10.1111/j.1365-2249.2004.02415.x. [ PMC free article ] [ PubMed ] [ CrossRef ] [ Google Scholar ]
- World Health Organization (2006) SARS: how a global epidemic was stopped. Manila: WHO Regional Office for the Western Pacific. https://www.file:///D:/DS/suzana.david/Downloads/9290612134_eng%20(1).pdf
- World Health Organization, WHO March 11 (2020) WHO Director-General's opening remarks at the media briefing on COVID-19—11 March 2020. https://www.who.int/dg/speeches/detail/who-director-general-s-opening-remarks-at-the-media-briefing-on-covid-19-11-march-2020 . Accessed 20 Dec 2021. https://www.who.int/docs/default-source/coronaviruse/transcripts/who-audio-emergencies-coronavirus-press-conference-full-and-final-11mar2020.pdf
- World Health organization, WHO November 17 (2020) Weekly epidemiological update - 17 November 2020. https://www.file:///D:/DS/suzana.david/Downloads/weekly-epi-update-14.pdf
- World Health Organization, WHO January 20 (2021) SARS-CoV-2 in animals used for fur farming: GLEWS+ risk assessment 20 January 2021 (No WHO/2019-nCoV/fur_farming/risk_assessment/20211). World Health Organization. http://www.fao.org/3/cb3368en/cb3368en.pdf
- World Health Organization, WHO (2022) Tracking SARS-CoV-2 variants. https://www.who.int/en/activities/tracking-SARS-CoV-2-variants/ . Accessed 26 Feb 2022
- World Health Organization, WHO (2020) The potential impact of health service disruptions on the burden of malaria, a modelling analysis for countries in sub-Saharan Africa. https://www.apps.who.int/iris/bitstream/handle/10665/331845/9789240004641-eng.pdf
- Wu F, Zhao S, Yu B, et al. A new coronavirus associated with human respiratory disease in China. Nature. 2020; 579 (7798):265–269. doi: 10.1038/s41586-020-2008-3. [ PMC free article ] [ PubMed ] [ CrossRef ] [ Google Scholar ]
- Wu J, Yuan X, Wang B, et al. Severe acute respiratory syndrome coronavirus 2: from gene structure to pathogenic mechanisms and potential therapy. Front Microbiol. 2020; 11 :1576. doi: 10.3389/fmicb.2020.01576. [ PMC free article ] [ PubMed ] [ CrossRef ] [ Google Scholar ]
- Xu Z, Shi L, Wang Y, et al. Pathological findings of COVID-19 associated with acute respiratory distress syndrome. Lancet Resp Med. 2020; 8 (4):420–422. doi: 10.1016/S2213-2600(20)30076-X. [ PMC free article ] [ PubMed ] [ CrossRef ] [ Google Scholar ]
- Yoneyama M, Fujita T. RNA recognition and signal transduction by RIG-I-like receptors. Immunol Rev. 2009; 227 (1):54–65. doi: 10.1111/j.1600-065X.2008.00727.x. [ PubMed ] [ CrossRef ] [ Google Scholar ]
- Yu P, Zhu J, Zhang Z, Han Y. A familial cluster of infection associated with the 2019 novel coronavirus indicating possible person-to-person transmission during the incubation period. J Infect Dis. 2020; 221 (11):1757–1761. doi: 10.1093/infdis/jiaa077. [ PMC free article ] [ PubMed ] [ CrossRef ] [ Google Scholar ]
- Zhao J, Zhao J, Perlman S. T cell responses are required for protection from clinical disease and for virus clearance in severe acute respiratory syndrome coronavirus-infected mice. J Virol. 2010; 84 (18):9318–9325. doi: 10.1128/JVI.01049-10. [ PMC free article ] [ PubMed ] [ CrossRef ] [ Google Scholar ]
- Zhao J, Zhao J, Mangalam AK, et al. Airway memory CD4+ T cells mediate protective immunity against emerging respiratory coronaviruses. Immunity. 2016; 44 (6):1379–1391. doi: 10.1016/j.immuni.2016.05.006. [ PMC free article ] [ PubMed ] [ CrossRef ] [ Google Scholar ]
- Zhao J, Yang Y, Huang H, et al. Relationship between the ABO blood group and the coronavirus disease 2019 (COVID-19) susceptibility. Clin Infect Dis. 2021; 73 (2):328–331. doi: 10.1093/cid/ciaa1150. [ PMC free article ] [ PubMed ] [ CrossRef ] [ Google Scholar ]
- Zhang X, Tan Y, Ling Y, et al. Viral and host factors related to the clinical outcome of COVID-19. Nature. 2020; 583 :437–440. doi: 10.1038/s41586-020-2355-0. [ PubMed ] [ CrossRef ] [ Google Scholar ]
- Zhang Q, Bastard P, Liu Z et al (2020b) Inborn errors of type I IFN immunity in patients with life-threatening COVID-19. Science 370(6515):eabd4570 [ PMC free article ] [ PubMed ]
- Zhang Y, Qin L, Zhao Y, Zhang P, Xu B, Li K, et al. Interferon-induced transmembrane protein 3 genetic variant rs12252-C associated with disease severity in coronavirus disease 2019. J Infect Dis. 2020; 222 (1):34–37. doi: 10.1093/infdis/jiaa224. [ PMC free article ] [ PubMed ] [ CrossRef ] [ Google Scholar ]
- Zheng M, Gao Y, Wang G, et al. Functional exhaustion of antiviral lymphocytes in COVID-19 patients. Cell Mol Immunol. 2020; 17 (5):533–535. doi: 10.1038/s41423-020-0402-2. [ PMC free article ] [ PubMed ] [ CrossRef ] [ Google Scholar ]
- Zheng HY, Zhang M, Yang CX, et al. Elevated exhaustion levels and reduced functional diversity of T cells in peripheral blood may predict severe progression in COVID-19 patients. Cell Mol Immunol. 2020; 17 (5):541–543. doi: 10.1038/s41423-020-0401-3. [ PMC free article ] [ PubMed ] [ CrossRef ] [ Google Scholar ]
- Zhou P, Yang XL, Wang XG, et al. A pneumonia outbreak associated with a new coronavirus of probable bat origin. Nature. 2020; 579 (7798):270–273. doi: 10.1038/s41586-020-2012-7. [ PMC free article ] [ PubMed ] [ CrossRef ] [ Google Scholar ]
- Zhou W, Wang W. Fast-spreading SARS-CoV-2 variants: challenges to and new design strategies of COVID-19 vaccines. Signal Transduct Target Ther. 2021; 6 :1–6. doi: 10.1038/s41392-021-00644-x. [ PMC free article ] [ PubMed ] [ CrossRef ] [ Google Scholar ]
- Zhu Y, Feng F, Hu G, et al. A genome-wide CRISPR screen identifies host factors that regulate SARS-CoV-2 entry. Nat Commun. 2021; 12 (1):1–11. [ PMC free article ] [ PubMed ] [ Google Scholar ]
- Zondervan KT, Cardon LR. Designing candidate gene and genome-wide case–control association studies. Nat Protoc. 2007; 2 (10):2492–2501. doi: 10.1038/nprot.2007.366. [ PMC free article ] [ PubMed ] [ CrossRef ] [ Google Scholar ]
- Zunec R. A review of HLA and COVID-19 association studies. Mol Exp Biol Med. 2020; 3 (2):25–30. doi: 10.33602/mebm.3.2.3. [ CrossRef ] [ Google Scholar ]
- About This IR
- Scholar Commons
Advancing Education in Quantitative Literacy
Home > Open Access Journals > NUMERACY > Vol. 14 (2021) > Iss. 1
Using COVID-19 Vaccine Efficacy Data to Teach One-Sample Hypothesis Testing
Frank Wang , LaGuardia Community College, CUNY Follow
COVID-19, hypothesis testing, Bayes’s rule, quantitative reasoning
In late November 2020, there was a flurry of media coverage of two companies’ claims of 95% efficacy rates of newly developed COVID-19 vaccines, but information about the confidence interval was not reported. This paper presents a way of teaching the concept of hypothesis testing and the construction of confidence intervals using numbers announced by the drug makers Pfizer and Moderna publicized by the media. Instead of a two-sample test or more complicated statistical models, we use the elementary one-proportion z -test to analyze the data. The method is designed to be accessible for students who have only taken a one-semester elementary statistics course. We will justify the use of a z -distribution as an approximation for the confidence interval of the efficacy rate. Bayes’s rule will be applied to relate the probability of being in the vaccine group among the volunteers who were infected by COVID-19 to the more consequential probability of being infected by COVID-19 given that the person is vaccinated.
https://doi.org/10.5038/1936-4660.14.1.1383
Recommended Citation
Wang, Frank. "Using COVID-19 Vaccine Efficacy Data to Teach One-Sample Hypothesis Testing." Numeracy 14, Iss. 1 (2021): Article 7. DOI: https://doi.org/10.5038/1936-4660.14.1.1383
Creative Commons License
Since January 11, 2021
Included in
Clinical Trials Commons , Higher Education and Teaching Commons
- Journal Home
- About This Journal
- Editorial Board
- Instructions to Authors
- Ethics and Malpractice Statement
- Submit Article
- Most Popular Papers
- Receive Email Notices or RSS
Advanced Search
ISSN: 1936-4660 (Online)
Scholar Commons | About This IR | FAQ | My Account | Accessibility Statement
Privacy Copyright
Log in using your username and password
- Search More Search for this keyword Advanced search
- Latest content
- Supplements
- BMJ Journals More You are viewing from: Google Indexer
You are here
- Volume 5, Issue 6
- Hypothesis to explain the severe form of COVID-19 in Northern Italy
- Article Text
- Article info
- Citation Tools
- Rapid Responses
- Article metrics

- http://orcid.org/0000-0002-6285-7355 Luca Cegolon 1 , 2 ,
- Jennifer Pichierri 3 ,
- Giuseppe Mastrangelo 4 ,
- Sandro Cinquetti 1 ,
- Giovanni Sotgiu 5 ,
- Saverio Bellizzi 6 ,
- Giuseppe Pichierri 7
- 1 Public Health Department , Local Health Unit N. 2 "Marca Trevigiana" , Treviso , Veneto Region , Italy
- 2 IRCCS Materno Infantile Burlo Garofolo , Trieste , Friuli-Venezia Giulia Region , Italy
- 3 Children and Young People’s Diabetes , University College London Hospitals NHS Foundation Trust , London , UK
- 4 Cardiac, Thoracic and Vascular Sciences , Padua University Hospital , Padua , Veneto Region , Italy
- 5 Department of Medical, Surgical and Experimental Sciences , University of Sassari , Sassari , Sardinia Region , Italy
- 6 Partnership for Maternal, Newborn and Child Health , World Health Organization , Geneve , Switzerland
- 7 Microbiology Department , Kingston Hospital NHS Foundation Trust , Kingston upon Thames , London , UK
- Correspondence to Dr Luca Cegolon; l.cegolon{at}gmail.com
https://doi.org/10.1136/bmjgh-2020-002564
Statistics from Altmetric.com
Request permissions.
If you wish to reuse any or all of this article please use the link below which will take you to the Copyright Clearance Center’s RightsLink service. You will be able to get a quick price and instant permission to reuse the content in many different ways.
- public health
- prevention strategies
- epidemiology
Summary box
What is already known about this subject.
Human coronaviruses are known to cause respiratory re-infections, regardless of pre-existing humoural immunity.
There is evidence suggesting that severe acute respiratory syndrome coronavirus type 2 (SARS-CoV-2) had been circulating in Italy before the first COVID-19 case was detected in the country.
What are the new findings?
Prior infections with SARS-CoV-2 (or other viruses/coronaviruses) may arguably predispose to more severe forms of the disease following re-infection with SARS-CoV-2, with an immunological mechanism known as Antibody-Dependent-Enhancement, already observed with infections sustained by other coronaviruses (MERS-CoV and SARS-CoV) or other viruses such as the West Nile Virus and Dengue.
What are the recommendations for policy and practice?
If confirmed by in vivo studies, this hypothesis may have relevant implications for the treatment of severe forms of COVID-19, yet the possibility to produce an effective vaccine against SARS-CoV-2 might be hampered.
The ongoing COVID-19 pandemic, caused by the novel severe acute respiratory syndrome coronavirus type 2 (SARS-CoV-2), has affected 212 countries worldwide at various degrees as of 8 May 2020. 1
In this paper we discuss a hypothesis that prior viral infections—either by SARS-CoV-2 or different strains of coronaviruses, or potentially even other respiratory viruses—may predispose to more severe forms of COVID-19, following a secondary infection with SARS-CoV-2.
Most COVID-19 infections are asymptomatic or manifest with mild to moderate respiratory symptoms (fever, cough, sore throat, myalgia, fatigue and even non-severe pneumonia). Of patients with COVID-19, 14%–15% develop severe pneumonia and 5%–6% a critical condition requiring admission to intensive care unit (ICU). 2–4 Death may eventually occur after an average of 17.8 days since the onset of symptoms. 5
Among all countries, Italy (which was the first European COVID-19 cluster) presents a critical disease pattern as of 8 May 2020, having the third highest number of COVID-19 cases in the world after the USA and Spain, the fourth highest prevalence of the disease after Spain, Belgium and the USA, the third highest total number of deaths attributed to COVID-19 after the USA and the UK, and the third highest prevalence of COVID-19 mortality after Belgium and Spain, despite a current 1% rate of severe/critical disease among active cases, which has been progressively decreasing over time. 1
The cross-country discrepancies in the burden of COVID-19 observed so far across the globe cannot be explained only by differences in population age structures. 6–8 In fact, Japan has a population double that of Italy, with the proportion of subjects older than 65 being 28.8% in Japan vs 21.7% in Italy. 9 10 Nonetheless, as of 8 May 2020, the difference in COVID-19 prevalence between Japan (122 per million) and Italy (3570 per million) is massive. 1 Likewise, in Germany the percentage of individuals >65 is reportedly 22.1% (hence slightly higher than Italy), but the prevalence of COVID-19 is currently 2022 per million. 1 11 In Iran the proportion of people >65 is 5.5% (hence much younger than the Italian, German and Japanese populations), but the prevalence of COVID-19 is 1246 per million, as of 8 May 2020. 1 12
The mortality rate for COVID-19 is reportedly enhanced by 5.6%–10.5% in the presence of any comorbidities (hypertension, diabetes, cardiovascular diseases, cancer and/or chronic respiratory conditions) and becomes significantly and progressively higher after 50 years of age, 4 6 although the severe form of the disease increases linearly at any age stage. 5 Cold dry weather is a recognised risk factor for respiratory infections, rendering viruses as influenza more stable and individuals more susceptible. 13 14 This applies also to SARS-CoV-2, the viability and transmissibility of which reportedly reduce with hot and humid weather conditions. 14 Moreover, unfavourable disease progression and clinical outcomes of COVID-19 were found to be associated with cigarette smoking in a systematic review. 15
A number of factors may have contributed to enhancing the risk of infection with SARS-CoV-2 in Northern Italy. The age by which half of all young people leave parental home is higher in Italy than other European Union countries, 16 and such multigenerational cohabitation probably contributed to increase COVID-19 contagion among elderly individuals. The universal use of face masks was initially discouraged in Italy in order to preserve the limited supplies of personal protective equipment for professional use in healthcare settings; another argument initially was that face masks are ineffective in protecting against coronavirus infections. 17 Further major findings of the relevant literature have been summarised in figure 1 . An extraordinary elevated incidence of COVID-19 could have been the result of a perfect storm triggered by multiple interplaying factors. The affected areas in Northern Italy (regions of Lombardy, Emilia-Romagna, Piedmont and Veneto) are characterised by high population density 18 and recognised air pollution, 19 20 especially from fine particulate matter (PM2.5), which was found to increase the risk of death from COVID-19 in the USA. 21 Northern Italy includes several cities which, similarly to Philadelphia (USA) during the Spanish flu pandemic in 1918, 22 are historically important and densely populated, where social gatherings as well as business activities are certainly fundamental—the latter being vital to the economy of the entire country. These cultural and social dynamics might have influenced the initial resistance and reluctance of the general population to comply with the social restrictions progressively enforced by the Italian government (until full lockdown was established on 21 March). Moreover, the intense case finding in Italy was preceded by an initial overall underestimation of the COVID-19 threat by the Italian government and subsequently by the general population, who perceived the disease as just some sort of influenza, despite worrying news from the first affected country (China). 23 Thereafter SARS-CoV-2 was also going to spread to other European countries, which have also now been heavily affected by the disease. 1
- Download figure
- Open in new tab
- Download powerpoint
Conceptual framework explaining the relationships between various factors and incidence and severe/critical form of COVID-19. Established items: orange boxes; hypothetical items: blue boxes. ADE, antibody-dependent enhancement; ARDS, acute respiratory distress syndrome; COVID-19, coronavirus infectious disease 2019; IPC, infection prevention and control; SARS-CoV-2, severe acute respiratory syndrome coronavirus type.
The epidemiological investigations conducted by the Italian National Institute of Health ( Istituto Superiore di Sanità ) suggest that the vast majority of cases but the first three acquired the infection in Italy. It can therefore be reasonably argued that SARS-CoV-2 had been circulating in the country—especially in Lombardy, Emilia-Romagna, Piedmont and Veneto regions—for weeks before the first patient was found. 24 Human coronaviruses are known to cause respiratory reinfections regardless of pre-existing humoural immunity, both at the individual and community level. 25 At the same time, presumed hospital-associated transmission of SARS-CoV-2 has been reported since the initial stages of the COVID-19 outbreak in Wuhan (China) in 41% of the total number of patients, 70% of whom were healthcare staff. 26 This could have also occurred in Italy, where healthcare workers reportedly make up 9% out of all COVID-19 cases. 27 We therefore hypothesise (see blue boxes in figure 1 ) that repeated cycles of infection within a community (especially in older adults)—or even more worryingly in a healthcare setting—could have the potential to cause more severe forms of COVID-19, with acute respiratory distress syndrome (ARDS), the fundamental pathophysiology of severe viral pneumonia due to COVID-19, requiring admission to ICU. 28 ARDS associated with COVID-19 shares clinical features with the critical form of the 2003 severe acute respiratory syndrome coronavirus (SARS-CoV) epidemic, in particular lymphopaenia, massive infiltration of phagocytes and inflammation sustained by cytokines. 4 8 29
A plausible mechanistic hypothesis could be the antibody-dependent enhancement (ADE), sustained by prior exposure to SARS-CoV-2 or other viruses/coronaviruses. 8 Previous circulation and exposure to other coronaviruses similar to SARS-CoV-2 causing mild/asymptomatic cold-like symptoms shall in fact not be ruled out. 8 Binding and neutralising antibody response against other types of human coronaviruses was recently reported to increase with age in adult patients, 25 and this may explain the increased linear risk of severe COVID-19 with age, with death being significantly higher in patients older than 50. 5
Non-neutralising, subneutralising or even fully neutralising antibodies may play a key role in ADE. 30 Wan et al 30 have recently described a molecular mechanism for ADE involving the Middle East respiratory syndrome coronavirus (MERS-CoV), similar to what is already known for SARS-CoV and flaviviruses as Dengue and the West Nile virus. 31–35 While the entry of SARS-CoV into the phagocytes occurred principally through the human ACE2 receptor, the ADE mechanism was shown to be enhanced by antibodies specific for the spike (S) envelope glycoprotein binding with the macrophage receptor and subsequent enhancement of target cell infections. 33–35 Likewise, the antibody/opsonised SARS-CoV-2 particles may bind avidly with the IgFc receptors of target cells, increasing the virus yield as well as the production of cytokines. This may also explain the higher risk of thromboembolism reportedly associated with severe/critical COVID-19. 4 36 37
Anecdotal clinical reports of ‘biphasic infection’ and ‘cytokine storm’ seem to possibly point towards this direction, biphasic infection simply being the immunological result of a secondary infection by other coronaviruses or a reinfection due to SARS-CoV-2. 38–41 An early elevation of serum proinflammatory cytokines, suggesting a pathological mechanism mediated by cytokine storm, has been observed with both severe forms of SARS-CoV and MERS-CoV infections. The latter two viruses share a genomic similarity of about 79% and 50% with SARS-CoV-2, respectively. 41 42 Several Rhinolophus bats-related coronavirus strains have been found to share even higher sequence homology with SARS-CoV-2. 33 An abnormal humoural response due to ADE, in the early stages of a secondary infection by SARS-CoV-2, may delay the innate antiviral immune response relying on the production of type 1 interferon (IFN-1). This would compromise the initial antiviral response of the host, with subsequent elevated influx of proinflammatory cytokines, hyperinflammatory neutrophils and monocytes-macrophages and hypercoagulable state accountable for ARDS and typical pneumonia observed in patients affected by severe/critical COVID-19 ( figure 1 ). 4 41 43 44
If confirmed, this hypothesis would have relevant implications for the treatment of COVID-19 and the development of an effective vaccine. The licensing of a vaccine against human coronaviruses has failed thus far, partly because immunised individuals could potentially be at higher risk of ADE sustained by facilitated uptake of viral antigen-antibody complexes by target cells. 4 31 33 44 The approval of a vaccine against SARS-CoV-2 may encounter similar obstacles. Likewise, herd immunity would not be a possibility with COVID-19. WHO recommends passive immunotherapy when vaccine and antivirals are not available for emerging infections. 45 In a preliminary uncontrolled case study on five critically ill patients with COVID-19 who developed ARDS, the administration of convalescent plasma—drawn from five patients who recovered from COVID-19 and containing SARS-CoV-2-specific neutralising antibodies (IgG)—between 10 and 22 days since admission significantly improved the clinical status of all, resolving ARDS in four of them within 12 days since transfusion. 46 On the other hand, the treatment of severe COVID-19 may also benefit from monoclonal antibodies targeting proinflammatory cytokines 4 as well as supplements of IFN-1 in combination with other antiviral drugs. 47–50
Whether secondary infections from other coronaviruses or repeated community reinfections of SARS-CoV-2 may account for more severe presentations of COVID-19 observed in some countries compared with others, 8 and whether it is only a matter of time for the virus to circulate and infect a significant proportion of the population before causing reinfections and therefore more severe clinical features, are something which will require more indepth epidemiological and immunological/serological investigations. A better understanding of any underlying immunological mechanism or any additional risk factor which could explain cross-country differences in the rates of severe disease and mortality attributable to COVID-19 will help to guide international public health responses during this ongoing pandemic. It will be important to clarify if the ARDS mechanism responsible for the severe respiratory infection could potentially be attributable to ADE.
Two different in vivo strategies could be employed to endorse this hypothesis.
First (observational design), all healthcare workers and blood donors should undergo serum test for COVID-19. Individuals presenting SARS-CoV-2 IgG antibodies should be included in a local/regional/national ad-hoc register and monitored over time for the possible development of severe disease sustained by ADE, which would need to be confirmed by blood count, dosage of IFN and proinflammatory cytokines, in addition to chest CT scan. The risk of developing severe COVID-19 should be estimated and stratified by relevant risk factors, including baseline serum level of SARS-CoV-2-specific IgG antibodies, age, sex, potential occupational exposure, medical history (particularly previous infections and vaccination status), any comorbidities, area of residence and health status of household members, among others.
Second (experimental laboratory design), animal models (hamsters, rodents, palm civets, monkeys, ferrets) 33 51 52 could be infected by SARS-CoV-2 (or other viruses/coronaviruses) and subsequently re-exposed to SARS-CoV-2 to verify the possibility of onset of ARDS sustained by ADE.
- Worldometers
- World Health Organization
- Chan JF-W ,
- Kok K-H , et al
- Dorigatti I , et al
- Index Mundi
- Feng K , et al
- Vardavas CI ,
- Iacovou M ,
- Xia N , et al
- Office for National Statistics (ISTAT)
- Ghermandi G ,
- Harrison RM
- Liang S , et al
- Nethery RC ,
- Sabath BM , et al
- Hannigan T ,
- Steele C , et al
- Start Magazine
- National Institute of Health (ISS)
- Donovan MM ,
- Hu C , et al
- International Council of Nurses
- Xu J , et al
- Cheung CY ,
- Ng IHY , et al
- Sun S , et al
- Gollins SW ,
- Porterfield JS
- Urbina AN ,
- Chang MR , et al
- Yang Z-yong ,
- Werner HC ,
- Kong W-pui , et al
- Tseng S-P ,
- Yen C-H , et al
- Tirado SMC ,
- Zhao L , et al
- Thachil J ,
- Iba T , et al
- McAuley DF ,
- Brown M , et al
- Mahallawi WH ,
- Khabour OF ,
- Zhang Q , et al
- Wu AKL , et al
- Prompetchara E ,
- Li J , et al
- Pedersen SF ,
- Corapi WV ,
- Ngichabe CK , et al
- Zhao F , et al
- Sallard E ,
- Lescure F-X ,
- Yazdanpanah Y , et al
- Chen Q , et al
- Loutfy MR ,
- Siminovitch KA , et al
- Omrani AS ,
- Baig K , et al
- Cheung CY , et al
- Roberts A , et al
Handling editor Seye Abimbola
Contributors LC, JP, SB, GP and GM equally contributed to conceiving the idea and drafting the manuscript. SC and GS contributed to drafting the manuscript.
Funding The authors have not declared a specific grant for this research from any funding agency in the public, commercial or not-for-profit sectors.
Competing interests None declared.
Patient consent for publication Not required.
Provenance and peer review Not commissioned; externally peer reviewed.
Data availability statement There are no data in this work.
Read the full text or download the PDF:

Testing environmental water to monitor COVID-19 spread in unsheltered encampments
To better understand COVID-19's spread during the pandemic, public health officials expanded wastewater surveillance. These efforts track SARS-CoV-2 levels and health risks among most people, but they miss people who live without shelter, a population particularly vulnerable to severe infection. To fill this information gap, researchers reporting in ACS' Environmental Science & Technology Letters tested flood-control waterways near unsheltered encampments, finding similar transmission patterns as in the broader community and identifying previously unseen viral mutations.
In recent years, testing untreated wastewater for SARS-CoV-2 incidence and dominant viral variants, as well as other pathogens, has been vital to helping public health officials determine infectious disease transmission in local communities. Yet, this monitoring only captures information on viruses shed from human feces and urine in buildings that are connected to local sewage infrastructure. Beyond the pandemic's impact on human health, it also exacerbated socioeconomic difficulties and increased the number of people experiencing homelessness and living in open-air encampments without access to indoor bathrooms. To understand the prevalence of COVID-19 among people who live unsheltered, Edwin Oh and colleagues tested for SARS-CoV-2 in waterways near encampments outside Las Vegas from December 2021 through July 2022.
Using quantitative polymerase chain reaction, the researchers identified SARS-CoV-2 RNA in more than 25% of the samples tested from two flood-control channels. The highest detection frequency over the study period aligned with Las Vegas' first wave of omicron variant infections, as confirmed through parallel testing at a local wastewater treatment plant. The researchers say these results suggest a similar level of transmission was occurring within the unsheltered community as it was among the general population. Then the researchers conducted whole genome sequencing to identify the SARS-CoV-2 variants in the waterways. These samples largely contained the same variants identified in the broader community. Deeper computational analysis of the viral sequences identified three novel viral spike protein mutations in some waterway samples, but the researchers have not yet examined what impact these mutations might have on viral function or clinical outcomes. Regardless, the ability to detect and identify SARS-CoV-2 in environmental water samples could help improve public health measures for a community that is often underrepresented in current surveillance methods. The researchers also say monitoring waterways could warn health officials of unexpected variants circulating in the community.
The authors acknowledge funding from the National Institutes of Health, the Nevada Governor's Office of Economic Development, the Centers for Disease Control and Prevention, and the Water Resources Research Institute of the United States Geological Survey.
- COVID and SARS
- Health Policy
- Environmental Policy
- Sustainability
- Public Health
- STEM Education
- Social Issues
- Public health
- Severe acute respiratory syndrome
- Epidemiology
- Yellow fever
- Spanish flu
Story Source:
Materials provided by American Chemical Society . Note: Content may be edited for style and length.
Journal Reference :
- Anthony Harrington, Van Vo, Michael A. Moshi, Ching-Lan Chang, Hayley Baker, Nabih Ghani, Jose Yani Itorralba, Katerina Papp, Daniel Gerrity, Duane Moser, Edwin C. Oh. Environmental Surveillance of Flood Control Infrastructure Impacted by Unsheltered Individuals Leads to the Detection of SARS-CoV-2 and Novel Mutations in the Spike Gene . Environmental Science & Technology Letters , 2024; DOI: 10.1021/acs.estlett.3c00938
Cite This Page :
Explore More
- Drug Development Made Easier
- RNA That Doesn't Age
- 'Rainbow' Detected On an Exoplanet
- Spears and Throwing Sticks 300,000 Years Old
- High Carbon Impact of Tourism at Yellowstone
- Extreme Starburst Galaxy
- Asthma: Disease May Be Stoppable
- Stellar Collisions and Zombie-Like Survivors
- Tiny Robot Swarms Inspired by Herd Mentality
- How the Brain Regulates Emotions
Trending Topics
Strange & offbeat.
COVID-19 Illness and Exposure Guidance
Updated April 4, 2024
Follow the instructions on the Public Health Flowchart for COVID-19 and Respiratory Virus Symptoms if you feel sick, test positive, or are exposed, and know when to report illness.
The University’s COVID-19 Health and Safety Plan contains additional requirements and guidance for reducing the risk of COVID-19 transmission in the workplace.
UW Medicine medical center employees and University personnel working in patient-facing clinics should consult their clinical supervisor for site-specific respiratory disease/COVID-19 policies and reporting requirements.

If you were exposed to a person with COVID-19
- You may continue normal activities (like returning to work and class) if you do not experience symptoms .
- Consider taking precautions to protect those around you, such as w earing a well-fitting mask or respirator when around others at home and in public for the next 5 days .
If you have any symptoms
- Stay home and away from others . Do not go to work or class.
- Take a test for COVID-19. Free rapid antigen tests are available on UW campuses.
- Wear a well-fitting mask or respirator if you must leave home for treatment or testing.
- Follow CDC guidance to prevent the spread of respiratory viruses.
If you test positive for COVID-19
- People who test positive are strongly encouraged to notify friends, colleagues, and relatives who may been exposed.
- If you are more likely to get very sick from COVID-19 (per CDC), consult your healthcare provider for isolation guidance, treatments available , and telehealth options that can reduce your chances of being hospitalized.
Returning to normal activities
You can go back to your normal activities after having COVID-19 or another respiratory illness when both conditions below are true:
- You are fever-free for 24 hours without the use of fever-reducing medication; and
- Your symptoms have improved.
Stay home i f you continue to have a fever, or if other symptoms have not improved (or worsened). Refer to the CDC examples to know how long to stay home.
When resuming normal activities, take added precautions for the next 5 days such as:
- Wear a face covering around others to prevent the spread of respiratory viruses. Masks can be picked up for free at various campus locations .
- Take a test when you may be around others with risk factors for severe illness or indoors. Tests can be picked up for free at various campus locations.
- Practice good sanitation and hygiene such as covering coughs and sneezes, washing your hands often, and cleaning common surfaces.
- When possible, open windows, gather outdoors, and use well-ventilated spaces .
- When possible, practice physical distancing between yourself and others.
- Stay up-to-date on immunizations recommended for you.
Reporting illness
UW personnel who believe their COVID-19 illness was a result of a workplace exposure are required to submit an incident report (OARS) to Environmental Health & Safety (EH&S) for follow up. Learn more about incident reporting .
If a supervisor believes personnel under their supervision are experiencing an outbreak of COVID-19 or respiratory illness (more than 10% of personnel are out sick) notify EH&S .
UW Medicine responds to COVID-19 cases involving personnel at UW Medicine medical facilities.
Notifications and privacy
Individuals who test positive for COVID-19 are strongly encouraged to let their close contacts know about their positive test.
If an individual who tests positive for COVID-19 chooses to notify EH&S, the identity of individuals who are suspected or confirmed to have COVID-19 and their close contacts is protected and is disclosed only on a need-to-know basis to those involved in the public health response. This may include UW Human Resources representatives, supervisors, and department or unit leadership.
More information
EH&S COVID-19 Health and Safety Resources
Washington State Department of Labor and Industries (L&I) Requirements and Guidance for Preventing COVID-19
Washington State Department of Health COVID-19 Guidelines
End of the Federal COVID-19 Public Health Emergency (PHE) Declaration
Public Health - Seattle & King County
Tacoma-Pierce County Public Health
Contact EH&S at [email protected] or (206) 616-3344 .
UW medical center personnel may contact their employee health services .
COVID-19 Campus Support Contact
Texas man sentenced to 7 years in prison for $7 million COVID-19 testing fraud scheme, officials say
A n Irving man was sentenced to seven years in prison for his role in a $7 million COVID-19 testing fraud, federal officials announced Thursday.
Terrance Barnard, 40, was indicted in December 2022 and pleaded guilty in September 2023 to conspiracy to commit health care fraud and aggravated identity theft. Barnard was sentenced Wednesday, DOJ officials said in a release, and a judge ordered Barnard to pay more than $7 million in restitution.
“These defendants took advantage of unsuspecting patients – and a global pandemic – to steal millions of dollars from insurers using private patient information,” said U.S. Attorney Leigha Simonton. “This sort of crime breaches patients’ trust and raises the cost of healthcare for all – at a time when access to medical care feels precarious to many.”
According to plea papers, Barnard admitted that he and his co-conspirators accessed private patient information – including names, dates of birth, and insurance subscriber numbers – through various clinics where Barnard worked as a contract lab technician. Barnard admitted that on some occasions, he took photographs of patient information and stored the images on “burner” phones, and on other occasions, he and a co-conspirator accessed the clinics’ confidential electronic medical records to obtain large amounts of patient information at once.
According to the DOJ, Barnard and his co-conspirators then used the patient information to submit claims to insurance providers – including Blue Cross Blue Shield, Cigna, United Healthcare, Aetna, Humana, and Molina Health Care – for COVID-19 testing that was never performed.
Barnard admitted that the “labs” at which the co-conspirators claimed the testing occurred, including TC Diagnostics, ME Diagnostics, and PHR Diagnostics, were, in fact, shell entities that never operated as labs. Collectively, these three entities submitted approximately $30 million in claims and were paid more than $7 million in reimbursements for fake testing.
Under the terms of his plea agreement, Barnard agreed to forfeit almost $2.5 million from bank accounts tied to him or his family, several real properties, five vehicles, and five luxury watches, according to DOJ's release.
The following people were also charged in the scheme:
- Connie Jo Clampitt
- William Paul Gray
All three pleaded guilty to their respective charges. Gray was sentenced to 54 months in prison for conspiracy to commit healthcare fraud, and Clampitt and Hogg await sentencing on April 10 and May 1, respectively.
More Texas headlines:
Thank you for visiting nature.com. You are using a browser version with limited support for CSS. To obtain the best experience, we recommend you use a more up to date browser (or turn off compatibility mode in Internet Explorer). In the meantime, to ensure continued support, we are displaying the site without styles and JavaScript.
- View all journals
- Explore content
- About the journal
- Publish with us
- Sign up for alerts
- Review Article
- Published: 13 January 2023
Long COVID: major findings, mechanisms and recommendations
- Hannah E. Davis ORCID: orcid.org/0000-0002-1245-2034 1 ,
- Lisa McCorkell ORCID: orcid.org/0000-0002-3261-6737 2 ,
- Julia Moore Vogel ORCID: orcid.org/0000-0002-4902-3540 3 &
- Eric J. Topol ORCID: orcid.org/0000-0002-1478-4729 3
Nature Reviews Microbiology volume 21 , pages 133–146 ( 2023 ) Cite this article
1.34m Accesses
940 Citations
16591 Altmetric
Metrics details
- Research data
- Viral infection
An Author Correction to this article was published on 17 April 2023
This article has been updated
Long COVID is an often debilitating illness that occurs in at least 10% of severe acute respiratory syndrome coronavirus 2 (SARS-CoV-2) infections. More than 200 symptoms have been identified with impacts on multiple organ systems. At least 65 million individuals worldwide are estimated to have long COVID, with cases increasing daily. Biomedical research has made substantial progress in identifying various pathophysiological changes and risk factors and in characterizing the illness; further, similarities with other viral-onset illnesses such as myalgic encephalomyelitis/chronic fatigue syndrome and postural orthostatic tachycardia syndrome have laid the groundwork for research in the field. In this Review, we explore the current literature and highlight key findings, the overlap with other conditions, the variable onset of symptoms, long COVID in children and the impact of vaccinations. Although these key findings are critical to understanding long COVID, current diagnostic and treatment options are insufficient, and clinical trials must be prioritized that address leading hypotheses. Additionally, to strengthen long COVID research, future studies must account for biases and SARS-CoV-2 testing issues, build on viral-onset research, be inclusive of marginalized populations and meaningfully engage patients throughout the research process.
Similar content being viewed by others
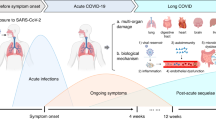
The long-term health outcomes, pathophysiological mechanisms and multidisciplinary management of long COVID
Jingwei Li, Yun Zhou, … Chengdi Wang
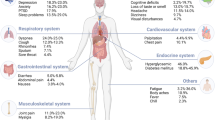
Epidemiology, clinical presentation, pathophysiology, and management of long COVID: an update
Sizhen Su, Yimiao Zhao, … Lin Lu
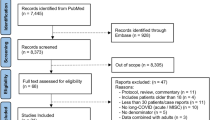
Long-COVID in children and adolescents: a systematic review and meta-analyses
Sandra Lopez-Leon, Talia Wegman-Ostrosky, … Sonia Villapol
Introduction
Long COVID (sometimes referred to as ‘post-acute sequelae of COVID-19’) is a multisystemic condition comprising often severe symptoms that follow a severe acute respiratory syndrome coronavirus 2 (SARS-CoV-2) infection. At least 65 million individuals around the world have long COVID, based on a conservative estimated incidence of 10% of infected people and more than 651 million documented COVID-19 cases worldwide 1 ; the number is likely much higher due to many undocumented cases. The incidence is estimated at 10–30% of non-hospitalized cases, 50–70% of hospitalized cases 2 , 3 and 10–12% of vaccinated cases 4 , 5 . Long COVID is associated with all ages and acute phase disease severities, with the highest percentage of diagnoses between the ages of 36 and 50 years, and most long COVID cases are in non-hospitalized patients with a mild acute illness 6 , as this population represents the majority of overall COVID-19 cases. There are many research challenges, as outlined in this Review, and many open questions, particularly relating to pathophysiology, effective treatments and risk factors.
Hundreds of biomedical findings have been documented, with many patients experiencing dozens of symptoms across multiple organ systems 7 (Fig. 1 ). Long COVID encompasses multiple adverse outcomes, with common new-onset conditions including cardiovascular, thrombotic and cerebrovascular disease 8 , type 2 diabetes 9 , myalgic encephalomyelitis/chronic fatigue syndrome (ME/CFS) 10 , 11 and dysautonomia, especially postural orthostatic tachycardia syndrome (POTS) 12 (Fig. 2 ). Symptoms can last for years 13 , and particularly in cases of new-onset ME/CFS and dysautonomia are expected to be lifelong 14 . With significant proportions of individuals with long COVID unable to return to work 7 , the scale of newly disabled individuals is contributing to labour shortages 15 . There are currently no validated effective treatments.
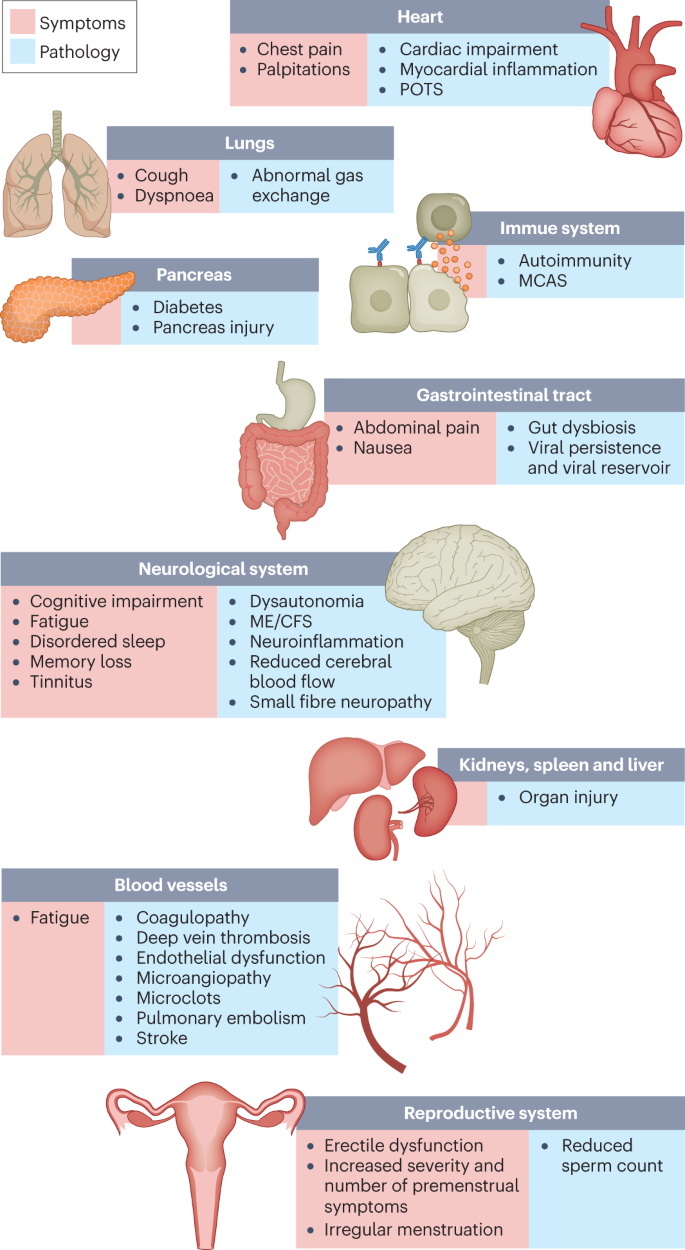
The impacts of long COVID on numerous organs with a wide variety of pathology are shown. The presentation of pathologies is often overlapping, which can exacerbate management challenges. MCAS, mast cell activation syndrome; ME/CFS, myalgic encephalomyelitis/chronic fatigue syndrome; POTS, postural orthostatic tachycardia syndrome.

Because diagnosis-specific data on large populations with long COVID are sparse, outcomes from general infections are included and a large proportion of medical conditions are expected to result from long COVID, although the precise proportion cannot be determined. One year after the initial infection, severe acute respiratory syndrome coronavirus 2 (SARS-CoV-2) infections increased the risk of cardiac arrest, death, diabetes, heart failure, pulmonary embolism and stroke, as studied with use of US Department of Veterans Affairs databases. Additionally, there is clear increased risk of developing myalgic encephalomyelitis/chronic fatigue syndrome (ME/CFS) and dysautonomia. Six months after breakthrough infection, increased risks were observed for cardiovascular conditions, coagulation and haematological conditions, death, fatigue, neurological conditions and pulmonary conditions in the same cohort. The hazard ratio is the ratio of how often an event occurs in one group relative to another; in this case people who have had COVID-19 compared with those who have not. Data sources are as follows: diabetes 9 , cardiovascular outcomes 8 , dysautonomia 12 , 201 , ME/CFS 10 , 202 and breakthrough infections 4 .
There are likely multiple, potentially overlapping, causes of long COVID. Several hypotheses for its pathogenesis have been suggested, including persisting reservoirs of SARS-CoV-2 in tissues 16 , 17 ; immune dysregulation 17 , 18 , 19 , 20 with or without reactivation of underlying pathogens, including herpesviruses such as Epstein–Barr virus (EBV) and human herpesvirus 6 (HHV-6) among others 17 , 18 , 21 , 22 ; impacts of SARS-CoV-2 on the microbiota, including the virome 17 , 23 , 24 , 25 ; autoimmunity 17 , 26 , 27 , 28 and priming of the immune system from molecular mimicry 17 ; microvascular blood clotting with endothelial dysfunction 17 , 29 , 30 , 31 ; and dysfunctional signalling in the brainstem and/or vagus nerve 17 , 32 (Fig. 3 ). Mechanistic studies are generally at an early stage, and although work that builds on existing research from postviral illnesses such as ME/CFS has advanced some theories, many questions remain and are a priority to address. Risk factors potentially include female sex, type 2 diabetes, EBV reactivation, the presence of specific autoantibodies 27 , connective tissue disorders 33 , attention deficit hyperactivity disorder, chronic urticaria and allergic rhinitis 34 , although a third of people with long COVID have no identified pre-existing conditions 6 . A higher prevalence of long Covid has been reported in certain ethnicities, including people with Hispanic or Latino heritage 35 . Socio-economic risk factors include lower income and an inability to adequately rest in the early weeks after developing COVID-19 (refs. 36 , 37 ). Before the emergence of SARS-CoV-2, multiple viral and bacterial infections were known to cause postinfectious illnesses such as ME/CFS 17 , 38 , and there are indications that long COVID shares their mechanistic and phenotypic characteristics 17 , 39 . Further, dysautonomia has been observed in other postviral illnesses and is frequently observed in long COVID 7 .
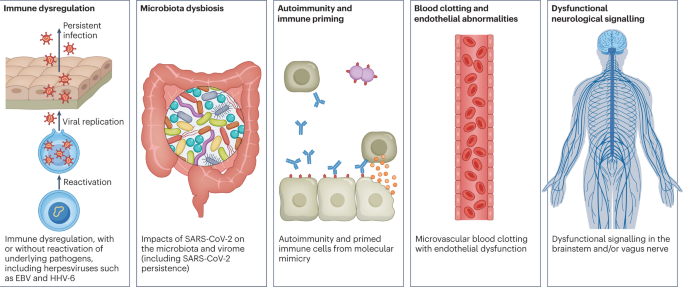
There are several hypothesized mechanisms for long COVID pathogenesis, including immune dysregulation, microbiota disruption, autoimmunity, clotting and endothelial abnormality, and dysfunctional neurological signalling. EBV, Epstein–Barr virus; HHV-6, human herpesvirus 6; SARS-CoV-2, severe acute respiratory syndrome coronavirus 2.
In this Review, we explore the current knowledge base of long COVID as well as misconceptions surrounding long COVID and areas where additional research is needed. Because most patients with long COVID were not hospitalized for their initial SARS-CoV-2 infection 6 , we focus on research that includes patients with mild acute COVID-19 (meaning not hospitalized and without evidence of respiratory disease). Most of the studies we discuss refer to adults, except for those in Box 1 .
Box 1 Long COVID in children
Long COVID impacts children of all ages. One study found that fatigue, headache, dizziness, dyspnoea, chest pain, dysosmia, dysgeusia, reduced appetite, concentration difficulties, memory issues, mental exhaustion, physical exhaustion and sleep issues were more common in individuals with long COVID aged 15–19 years compared with controls of the same age 203 . A nationwide study in Denmark comparing children with a positive PCR test result with control individuals found that the former had a higher chance of reporting at least one symptom lasting more than 2 months 204 . Similarly to adults with long COVID, children with long COVID experience fatigue, postexertional malaise, cognitive dysfunction, memory loss, headaches, orthostatic intolerance, sleep difficulty and shortness of breath 204 , 205 . Liver injury has been recorded in children who were not hospitalized during acute severe acute respiratory syndrome coronavirus 2 (SARS-CoV-2) infections 206 , and although rare, children who had COVID-19 have increased risks of acute pulmonary embolism, myocarditis and cardiomyopathy, venous thromboembolic events, acute and unspecified renal failure, and type 1 diabetes 207 . Infants born to women who had COVID-19 during pregnancy were more likely to receive a neurodevelopmental diagnosis in the first year after delivery 208 . A paediatric long COVID centre’s experience treating patients suggests that adolescents with a moderate to severe form of long COVID have features consistent with myalgic encephalomyelitis/chronic fatigue syndrome 205 . Children experiencing long COVID have hypometabolism in the brain similar to the patterns found in adults with long COVID 209 . Long-term pulmonary dysfunction is found in children with long COVID and those who have recovered from COVID-19 (ref. 210 ). Children with long COVID were more likely to have had attention deficit hyperactivity disorder, chronic urticaria and allergic rhinitis before being infected 34 .
More research on long COVID in children is needed, although there are difficulties in ensuring a proper control group due to testing issues. Several studies have found that children infected with SARS-CoV-2 are considerably less likely to have a positive PCR test result than adults despite seroconverting weeks later, with up to 90% of cases being missed 189 , 190 . Additionally, children are much less likely to seroconvert and, if they develop antibodies, are more likely to have a waning response months after infection compared with adults 193 .
Major findings
Immunology and virology.
Studies looking at immune dysregulation in individuals with long COVID who had mild acute COVID-19 have found T cell alterations, including exhausted T cells 18 , reduced CD4 + and CD8 + effector memory cell numbers 18 , 19 and elevated PD1 expression on central memory cells, persisting for at least 13 months 19 . Studies have also reported highly activated innate immune cells, a lack of naive T and B cells and elevated expression of type I and type III interferons (interferon-β (IFNβ) and IFNλ1), persisting for at least 8 months 20 . A comprehensive study comparing patients with long COVID with uninfected individuals and infected individuals without long COVID found increases in the numbers of non-classical monocytes, activated B cells, double-negative B cells, and IL-4- and IL-6-secreting CD4 + T cells and decreases in the numbers of conventional dendritic cells and exhausted T cells and low cortisol levels in individuals with long COVID at a median of 14 months after infection 18 . The expansion of cytotoxic T cells has been found to be associated with the gastrointestinal presentation of long COVID 27 . Additional studies have found elevated levels of cytokines, particularly IL-1β, IL-6, TNF and IP10 (refs. 40 , 41 ), and a recent preprint has reported persistent elevation of the level of CCL11, which is associated with cognitive dysfunction 42 . It remains to be seen whether the pattern of cytokines in ME/CFS, where the levels of certain cytokines are elevated in the first 2–3 years of illness but decrease over time without a corresponding decrease in symptoms 43 , is similar in long COVID.
Multiple studies have found elevated levels of autoantibodies in long COVID 27 , including autoantibodies to ACE2 (ref. 28 ) (the receptor for SARS-CoV-2 entry), β 2 -adrenoceptor, muscarinic M2 receptor, angiotensin II AT 1 receptor and the angiotensin 1–7 MAS receptor 26 . High levels of other autoantibodies have been found in some patients with COVID-19 more generally, including autoantibodies that target the tissue (such as connective tissue, extracellular matrix components, vascular endothelium, coagulation factors and platelets), organ systems (including the lung, central nervous system, skin and gastrointestinal tract), immunomodulatory proteins (cytokines, chemokines, complement components and cell-surface proteins) 44 . A major comprehensive study, however, did not find autoantibodies to be a major component of long COVID 18 .
Reactivated viruses, including EBV and HHV-6, have been found in patients with long COVID 18 , 21 , 22 , 27 (and have been identified in ME/CFS 45 ), and lead to mitochondrial fragmentation and severely affect energy metabolism 46 . A recent preprint has reported that EBV reactivation is associated with fatigue and neurocognitive dysfunction in patients with long COVID 22 .
Several studies have shown low or no SARS-CoV-2 antibody production and other insufficient immune responses in the acute stage of COVID-19 to be predictive of long COVID at 6–7 months, in both hospitalized patients and non-hospitalized patients 47 , 48 . These insufficient immune responses include a low baseline level of IgG 48 , low levels of receptor-binding domain and spike-specific memory B cells, low levels of nucleocapsid IgG 49 and low peaks of spike-specific IgG 47 . In a recent preprint, low or absent CD4 + T cell and CD8 + T cell responses were noted in patients with severe long COVID 49 , and a separate study found lower levels of CD8 + T cells expressing CD107a and a decline in nucleocapsid-specific interferon-γ-producing CD8 + T cells in patients with long COVID compared with infected controls without long COVID 50 . High levels of autoantibodies in long COVID have been found to be inversely correlated with protective COVID-19 antibodies, suggesting that patients with high autoantibody levels may be more likely to have breakthrough infections 27 . SARS-CoV-2 viral rebound in the gut, possibly resulting from viral persistence, has also been associated with lower levels and slower production of receptor-binding domain IgA and IgG antibodies 51 . There are major differences in antibody creation, seroreversion and antibody titre levels across the sexes, with women being less likely to seroconvert, being more likely to serorevert and having lower antibody levels overall 52 , 53 , even affecting antibody waning after vaccination 54 .
Several reports have pointed towards possible viral persistence as a driver of long COVID symptoms; viral proteins and/or RNA has been found in the reproductive system, cardiovascular system, brain, muscles, eyes, lymph nodes, appendix, breast tissue, hepatic tissue, lung tissue, plasma, stool and urine 55 , 56 , 57 , 58 , 59 , 60 . In one study, circulating SARS-CoV-2 spike antigen was found in 60% of a cohort of 37 patients with long COVID up to 12 months after diagnosis compared with 0% of 26 SARS-CoV-2-infected individuals, likely implying a reservoir of active virus or components of the virus 16 . Indeed, multiple reports following gastrointestinal biopsies have indicated the presence of virus, suggestive of a persistent reservoir in some patients 58 , 61 .
Vascular issues and organ damage
Although COVID-19 was initially recognized as a respiratory illness, SARS-CoV-2 has capability to damage many organ systems. The damage that has been demonstrated across diverse tissues has predominantly been attributed to immune-mediated response and inflammation, rather than direct infection of cells by the virus. Circulatory system disruption includes endothelial dysfunction and subsequent downstream effects, and increased risks of deep vein thrombosis, pulmonary embolism and bleeding events 29 , 30 , 62 . Microclots detected in both acute COVID-19 and long COVID contribute to thrombosis 63 and are an attractive diagnostic and therapeutic target. Long-term changes to the size and stiffness of blood cells have also been found in long COVID, with the potential to affect oxygen delivery 64 . A long-lasting reduction in vascular density, specifically affecting small capillaries, was found in patients with long COVID compared with controls, 18 months after infection 65 . A study finding elevated levels of vascular transformation blood biomarkers in long COVID also found that the angiogenesis markers ANG1 and P-selectin both had high sensitivity and specificity for predicting long COVID status 66 .
An analysis of the US Department of Veterans Affairs databases (VA data) including more than 150,000 individuals 1 year after SARS-CoV-2 infection indicated a significantly increased risk of a variety of cardiovascular diseases, including heart failure, dysrhythmias and stroke, independent of the severity of initial COVID-19 presentation 8 (Fig. 2 ). Cardiac MRI studies revealed cardiac impairment in 78% of 100 individuals who had a prior COVID-19 episode (investigated an average of 71 days after infection 67 ) and in 58% of participants with long COVID (studied 12 months after infection 68 ), reinforcing the durability of cardiac abnormalities.
Multiple studies have revealed multi-organ damage associated with COVID-19. One prospective study of low-risk individuals, looking at the heart, lungs, liver, kidneys, pancreas and spleen, noted that 70% of 201 patients had damage to at least one organ and 29% had multi-organ damage 69 . In a 1-year follow-up study, conducted by the same research group with 536 participants, the study authors found that 59% had single-organ damage and 27% multi-organ damage 70 . A dedicated kidney study of VA data including more than 89,000 individuals who had COVID-19 noted an increased risk of numerous adverse kidney outcomes 71 . Another VA data analysis, including more than 181,000 individuals who had COVID-19, found that infection also increases the risk of type 2 diabetes 9 (Fig. 2 ). The organ damage experienced by patients with long COVID appears durable, and long-term effects remain unknown.
Neurological and cognitive systems
Neurological and cognitive symptoms are a major feature of long COVID, including sensorimotor symptoms, memory loss, cognitive impairment, paresthesia, dizziness and balance issues, sensitivity to light and noise, loss of (or phantom) smell or taste, and autonomic dysfunction, often impacting activities of daily living 7 , 32 . Audiovestibular manifestations of long COVID include tinnitus, hearing loss and vertigo 7 , 72 .
In a meta-analysis, fatigue was found in 32% and cognitive impairment was found in 22% of patients with COVID-19 at 12 weeks after infection 3 . Cognitive impairments in long COVID are debilitating, at the same magnitude as intoxication at the UK drink driving limit or 10 years of cognitive ageing 73 , and may increase over time, with one study finding occurrence in 16% of patients at 2 months after infection and 26% of patients at 12 months after infection 74 . Activation of the kynurenine pathway, particularly the presence of the metabolites quinolinic acid, 3-hydroxyanthranilic acid and kynurenine, has been identified in long COVID, and is associated with cognitive impairment 74 . Cognitive impairment has also been found in individuals who recovered from COVID-19 (ref. 75 ), and at higher rates when objective versus subjective measures were used 3 , suggesting that a subset of those with cognitive impairment may not recognize and/or report their impairment. Cognitive impairment is a feature that manifests itself independently of mental health conditions such as anxiety and depression 74 , 76 , and occurs at similar rates in hospitalized and non-hospitalized patients 74 , 76 . A report of more than 1.3 million people who had COVID-19 showed mental health conditions such as anxiety and depression returned to normal over time, but increased risks of cognitive impairment (brain fog), seizures, dementia, psychosis and other neurocognitive conditions persisted for at least 2 years 77 .
Possible mechanisms for these neuropathologies include neuroinflammation, damage to blood vessels by coagulopathy and endothelial dysfunction, and injury to neurons 32 . Studies have found Alzheimer disease-like signalling in patients with long COVID 78 , peptides that self-assemble into amyloid clumps which are toxic to neurons 79 , widespread neuroinflammation 80 , brain and brainstem hypometabolism correlated with specific symptoms 81 , 82 and abnormal cerebrospinal fluid findings in non-hospitalized individuals with long COVID along with an association between younger age and a delayed onset of neurological symptoms 83 . Multilineage cellular dysregulation and myelin loss were reported in a recent preprint in patients with long COVID who had mild infections, with microglial reactivity similar to that seen in chemotherapy, known as ‘chemo-brain’ 42 . A study from the UK Biobank, including brain imaging in the same patients before and after COVID-19 as well as control individuals, showed a reduction in grey matter thickness in the orbitofrontal cortex and parahippocampal gyrus (markers of tissue damage in areas connected to the primary olfactory cortex), an overall reduction in brain size and greater cognitive decline in patients after COVID-19 compared with controls, even in non-hospitalized patients. Although that study looked at individuals with COVID-19 compared with controls, not specifically long COVID, it may have an implication for the cognitive component of long COVID 84 . Abnormal levels of mitochondrial proteins as well as SARS-CoV-2 spike and nucleocapsid proteins have been found in the central nervous system 85 . Tetrahydrobiopterin deficiencies and oxidative stress are found in long COVID as well 86 .
In the eyes, corneal small nerve fibre loss and increased dendritic cell density have been found in long COVID 87 , 88 , as well as significantly altered pupillary light responses 89 and impaired retinal microcirculation 90 . SARS-CoV-2 can infect and replicate in retinal 59 and brain 91 organoids. Other manifestations of long COVID include retinal haemorrhages, cotton wool spots and retinal vein occlusion 92 .
Mouse models of mild SARS-CoV-2 infection demonstrated microglial reactivity and elevated levels of CCL11, which is associated with cognitive dysfunction and impaired neurogenesis 42 . Hamster models exhibited an ongoing inflammatory state, involving T cell and myeloid activation, production of pro-inflammatory cytokines and an interferon response that was correlated with anxiety and depression-like behaviours in the hamsters, with similar transcriptional signatures found in the tissue of humans who had recovered from COVID-19 (ref. 93 ). Infected non-human primates with mild illness showed neuroinflammation, neuronal injury and apoptosis, brain microhaemorrhages, and chronic hypoxaemia and brain hypoxia 94 .
Recent reports indicate low blood cortisol levels in patients with long COVID as compared with control individuals, more than 1 year into symptom duration 18 , 27 . Low cortisol production by the adrenal gland should be compensated by an increase in adrenocorticotropic hormone (ACTH) production by the pituitary gland, but this was not the case, supporting hypothalamus–pituitary–adrenal axis dysfunction 18 . This may also reflect an underlying neuroinflammatory process. Low cortisol levels have previously been documented in individuals with ME/CFS.
ME/CFS, dysautonomia and related conditions
ME/CFS is a multisystem neuroimmune illness with onset often following a viral or bacterial infection. Criteria include a “substantial reduction or impairment in the ability to engage in pre-illness levels of occupational, educational, social, or personal activities” for at least 6 months, accompanied by a profound fatigue that is not alleviated by rest, along with postexertional malaise, unrefreshing sleep and cognitive impairment or orthostatic intolerance (or both) 95 . Up to 75% of people with ME/CFS cannot work full-time and 25% have severe ME/CFS, which often means they are bed-bound, have extreme sensitivity to sensory input and are dependent on others for care 96 . There is a vast collection of biomedical findings in ME/CFS 97 , 98 , although these are not well known to researchers and clinicians in other fields.
Many researchers have commented on the similarity between ME/CFS and long COVID 99 ; around half of individuals with long COVID are estimated to meet the criteria for ME/CFS 10 , 11 , 29 , 100 , and in studies where the cardinal ME/CFS symptom of postexertional malaise is measured, a majority of individuals with long COVID report experiencing postexertional malaise 7 , 100 . A study of orthostatic stress in individuals with long COVID and individuals with ME/CFS found similar haemodynamic, symptomatic and cognitive abnormalities in both groups compared with healthy individuals 101 . Importantly, it is not surprising that ME/CFS should stem from SARS-CoV-2 infection as 27.1% of SARS-CoV infection survivors in one study met the criteria for ME/CFS diagnosis 4 years after onset 102 . A wide range of pathogens cause ME/CFS onset, including EBV, Coxiella burnetii (which causes Q fever), Ross River virus and West Nile virus 38 .
Consistent abnormal findings in ME/CFS include diminished natural killer cell function, T cell exhaustion and other T cell abnormalities, mitochondrial dysfunction, and vascular and endothelial abnormalities, including deformed red blood cells and reduced blood volume. Other abnormalities include exercise intolerance, impaired oxygen consumption and a reduced anaerobic threshold, and abnormal metabolic profiles, including altered usage of fatty acids and amino acids. Altered neurological functions have also been observed, including neuroinflammation, reduced cerebral blood flow, brainstem abnormalities and elevated ventricular lactate level, as well as abnormal eye and vision findings. Reactivated herpesviruses (including EBV, HHV-6, HHV-7 and human cytomegalovirus) are also associated with ME/CFS 97 , 98 , 103 , 104 .
Many of these findings have been observed in long COVID studies in both adults and children (Box 1 ). Long COVID research has found mitochondrial dysfunction including loss of mitochondrial membrane potential 105 and possible dysfunctional mitochondrial metabolism 106 , altered fatty acid metabolism and dysfunctional mitochondrion-dependent lipid catabolism consistent with mitochondrial dysfunction in exercise intolerance 107 , redox imbalance 108 , and exercise intolerance and impaired oxygen extraction 100 , 109 , 110 . Studies have also found endothelial dysfunction 29 , cerebral blood flow abnormalities and metabolic changes 81 , 111 , 112 , 113 (even in individuals with long COVID whose POTS symptoms abate 114 ), extensive neuroinflammation 42 , 80 , reactivated herpesviruses 18 , 21 , 27 , deformed red blood cells 64 and many findings discussed elsewhere. Microclots and hyperactivated platelets are found not only in individuals with long COVID but also in individuals with ME/CFS 115 .
Dysautonomia, particularly POTS, is commonly comorbid with ME/CFS 116 and also often has a viral onset 117 . POTS is associated with G protein-coupled adrenergic receptor and muscarinic acetylcholine receptor autoantibodies, platelet storage pool deficiency, small fibre neuropathy and other neuropathologies 118 . Both POTS and small fibre neuropathy are commonly found in long COVID 111 , 119 , with one study finding POTS in 67% of a cohort with long COVID 120 .
Mast cell activation syndrome is also commonly comorbid with ME/CFS. The number and severity of mast cell activation syndrome symptoms substantially increased in patients with long COVID compared with pre-COVID and control individuals 121 , with histamine receptor antagonists resulting in improvements in the majority of patients 19 .
Other conditions that are commonly comorbid with ME/CFS include connective tissue disorders including Ehlers–Danlos syndrome and hypermobility, neuro-orthopaedic spinal and skull conditions, and endometriosis 33 , 122 , 123 . Evidence is indicating these conditions may be comorbid with long COVID as well. The overlap of postviral conditions with these conditions should be explored further.
Reproductive system
Impacts on the reproductive system are often reported in long COVID, although little research has been done to document the extent of the impact and sex-specific pathophysiology. Menstrual alterations are more likely to occur in women and people who menstruate with long COVID than in women and people who menstruate with no history of COVID and those who had COVID-19 but not long COVID 124 . Menstruation and the week before menstruation have been identified by patients as triggers for relapses of long COVID symptoms 7 . Declined ovarian reserve and reproductive endocrine disorder have been observed in people with COVID-19 (ref. 125 ), and initial theories suggest that SARS-CoV-2 infection affects ovary hormone production and/or the endometrial response due to the abundance of ACE2 receptors on ovarian and endometrial tissue 126 . Individuals with both COVID-19 and menstrual changes were more likely to experience fatigue, headache, body ache and pain, and shortness of breath than those who did not have menstrual changes, and the most common menstrual changes were irregular menstruation, increased premenstrual symptoms and infrequent menstruation 127 .
Research on ME/CFS shows associations between ME/CFS and premenstrual dysphoric disorder, polycystic ovarian syndrome, menstrual cycle abnormalities, ovarian cysts, early menopause and endometriosis 128 , 129 , 130 . Pregnancy, postpartum changes, perimenopause and menstrual cycle fluctuations affect ME/CFS and influence metabolic and immune system changes 129 . Long COVID research should focus on these relationships to better understand the pathophysiology.
Viral persistence in the penile tissue has been documented, as has an increased risk of erectile dysfunction, likely resulting from endothelial dysfunction 131 . In one study, impairments to sperm count, semen volume, motility, sperm morphology and sperm concentration were reported in individuals with long COVID compared with control individuals, and were correlated with elevated levels of cytokines and the presence of caspase 8, caspase 9 and caspase 3 in seminal fluid 132 .
Respiratory system
Respiratory conditions are a common phenotype in long COVID, and in one study occurred twice as often in COVID-19 survivors as in the general population 2 . Shortness of breath and cough are the most common respiratory symptoms, and persisted for at least 7 months in 40% and 20% of patients with long COVID, respectively 7 . Several imaging studies that included non-hospitalized individuals with long COVID demonstrated pulmonary abnormalities including in air trapping and lung perfusion 133 , 134 . An immunological and proteomic study of patients 3–6 months after infection indicated apoptosis and epithelial damage in the airway but not in blood samples 135 . Further immunological characterization comparing individuals with long COVID with individuals who had recovered from COVID-19 noted a correlation between decreased lung function, systemic inflammation and SARS-CoV-2-specific T cells 136 .
Gastrointestinal system
Long COVID gastrointestinal symptoms include nausea, abdominal pain, loss of appetite, heartburn and constipation 137 . The gut microbiota composition is significantly altered in patients with COVID-19 (ref. 23 ), and gut microbiota dysbiosis is also a key component of ME/CFS 138 . Higher levels of Ruminococcus gnavus and Bacteroides vulgatus and lower levels of Faecalibacterium prausnitzii have been found in people with long COVID compared with non-COVID-19 controls (from before the pandemic), with gut dysbiosis lasting at least 14 months; low levels of butyrate-producing bacteria are strongly correlated with long COVID at 6 months 24 . Persisting respiratory and neurological symptoms are each associated with specific gut pathogens 24 . Additionally, SARS-CoV-2 RNA is present in stool samples of patients with COVID-19 (ref. 139 ), with one study indicating persistence in the faeces of 12.7% of participants 4 months after diagnosis of COVID-19 and in 3.8% of participants at 7 months after diagnosis 61 . Most patients with long COVID symptoms and inflammatory bowel disease 7 months after infection had antigen persistence in the gut mucosa 140 . Higher levels of fungal translocation, from the gut and/or lung epithelium, have been found in the plasma of patients with long COVID compared with those without long COVID or SARS-CoV-2-negative controls, possibly inducing cytokine production 141 . Transferring gut bacteria from patients with long COVID to healthy mice resulted in lost cognitive functioning and impaired lung defences in the mice, who were partially treated with the commensal probiotic bacterium Bifidobacterium longum 25 .
The onset and time course of symptoms differ across individuals and by symptom type. Neurological symptoms often have a delayed onset of weeks to months: among participants with cognitive symptoms, 43% reported a delayed onset of cognitive symptoms at least 1 month after COVID-19, with the delay associated with younger age 83 . Several neurocognitive symptoms worsen over time and tend to persist longer, whereas gastrointestinal and respiratory symptoms are more likely to resolve 7 , 74 , 142 . Additionally, pain in joints, bones, ears, neck and back are more common at 1 year than at 2 months, as is paresthesia, hair loss, blurry vision and swelling of the legs, hands and feet 143 . Parosmia has an average onset of 3 months after the initial infection 144 ; unlike other neurocognitive symptoms, it often decreases over time 143 .
Few people with long COVID demonstrate full recovery, with one study finding that 85% of patients who had symptoms 2 months after the initial infection reported symptoms 1 year after symptom onset 143 . Future prognosis is uncertain, although diagnoses of ME/CFS and dysautonomia are generally lifelong.
Diagnostic tools and treatments
Although diagnostic tools exist for some components of long COVID (for example, tilt table tests for POTS 145 and MRI scans to detect cardiovascular impairment 68 ), diagnostic tools for long COVID are mostly in development, including imaging to detect microclots 63 , corneal microscopy to identify small fibre neuropathy 87 , new fragmentation of QRS complex on electrocardiograms as indicative of cardiac injury 146 and use of hyperpolarized MRI to detect pulmonary gas exchange abnormalities 147 . On the basis of the tests that are offered as standard care, the results for patients with long COVID are often normal; many providers are unaware of the symptom-specific testing and diagnostic recommendations from the ME/CFS community 148 . Early research into biomarkers suggests that levels of extracellular vesicles 85 and/or immune markers indicating high cytotoxicity 149 could be indicative of long COVID. Intriguingly, dogs can identify individuals with long COVID on the basis of sweat samples 150 . Biomarker research in ME/CFS may also be applicable to long COVID, including electrical impedance blood tests, saliva tests, erythrocyte deformation, sex-specific plasma lipid profiles and variables related to isocapnic buffering 151 , 152 , 153 , 154 . The importance of developing and validating biomarkers that can be used for the diagnosis of long COVID cannot be adequately emphasized — they will not only be helpful in establishing the diagnosis but will also be helpful for objectively defining treatment responses.
Although there are currently no broadly effective treatments for long COVID, treatments for certain components have been effective for subsets of populations (Table 1 ). Many strategies for ME/CFS are effective for individuals with long COVID, including pacing 7 , 37 and symptom-specific pharmacological options (for example, β-blockers for POTS, low-dose naltrexone for neuroinflammation 155 and intravenous immunoglobulin for immune dysfunction) and non-pharmacological options (including increasing salt intake for POTS, cognitive pacing for cognitive dysfunction and elimination diets for gastrointestinal symptoms) 96 . Low-dose naltrexone has been used in many diseases, including ME/CFS 155 , and has also shown promise in treating long COVID 156 . H 1 and H 2 antihistamines, often following protocols for mast cell activation syndrome and particularly involving famotidine, are used to alleviate a wide range of symptoms 19 , 157 , although they are not a cure. Another drug, BC007, potentially addresses autoimmunity by neutralizing G protein-coupled receptor autoantibody levels 158 . Anticoagulant regimens are a promising way to address abnormal clotting 159 ; in one study, resolution of symptoms was seen in all 24 patients receiving triple anticoagulant therapy 31 . Apheresis has also shown promise to alleviate long COVID symptoms; it has been theorized to help remove microclots 160 and has been shown to reduce autoantibodies in ME/CFS 161 . However, it is quite expensive, and its benefits are uncertain. Some supplements have shown promise in treating both long COVID and ME/CFS, including coenzyme Q 10 and d -ribose 162 , and may deserve further study.
Of note, exercise is harmful for patients with long COVID who have ME/CFS or postexertional malaise 110 , 163 and should not be used as a treatment 164 , 165 , 166 ; one study of people with long COVID noted that physical activity worsened the condition of 75% of patients, and less than 1% saw improvement 109 .
Pilot studies and case reports have revealed additional treatment options worth exploring. A case report noted resolution of long COVID following treatment with the antiviral Paxlovid 167 , and a study investigating the treatment of acute COVID-19 with Paxlovid showed a 25% reduction in the incidence of long COVID 168 ; Paxlovid should be investigated further for prevention and treatment of long COVID. A small trial of sulodexide in individuals with endothelial dysfunction saw a reduction in symptom severity 169 . Pilot studies of probiotics indicated potential in alleviating gastrointestinal and non-gastrointestinal symptoms 170 , 171 . Two patients with long COVID experienced substantial alleviation of dysautonomia symptoms following stellate ganglion block 172 . An early study noted that Pycnogenol statistically significantly improved physiological measurements (for example, reduction in oxidative stress) and quality of life (indicated by higher Karnofsky Performance Scale Index scores) 173 , 174 , as hypothesized on the basis of success in other clinical studies.
Taken together, the current treatment options are based on small-scale pilot studies in long COVID or what has been effective in other diseases; several additional trials are in progress 175 . There is a wide range of possible treatment options from ME/CFS covering various mechanisms, including improving natural killer cell function, removing autoantibodies, immunosuppressants, antivirals for reactivated herpesviruses, antioxidants, mitochondrial support and mitochondrial energy generation 176 , 177 ; most need to be clinically trialled, which should happen urgently. Many newer treatment options remain underexplored, including anticoagulants and SARS-CoV-2-specific antivirals, and a lack of funding is a significant limitation to robust trials.
Impact of vaccines, variants and reinfections
The impact of vaccination on the incidence of long COVID differs across studies, in part because of differing study methods, time since vaccination and definitions of long COVID. One study indicated no significant difference in the development of long COVID between vaccinated individuals and unvaccinated individuals 178 ; other studies indicate that vaccines provide partial protection, with a reduced risk of long COVID between 15% and 41% 4 , 5 , with long COVID continuing to impact 9% of people with COVID-19.
The different SARS-CoV-2 variants and level of (and time since) vaccination may impact the development of long COVID. The UK’s Office for National Statistics found that long COVID was 50% less common in double-vaccinated participants with Omicron BA.1 than in double-vaccinated participants Delta, but that there was no significant difference between triple-vaccinated participants; it also found long COVID was more common after Omicron BA.2 infection than after BA.1 infection in triple-vaccinated participants, with 9.3% developing long COVID from infection with the BA.2 variant 179 .
The impact of vaccination on long COVID symptoms in people who had already developed long COVID differs among patients, with 16.7% of patients experiencing a relief of symptoms, 21.4% experiencing a worsening of symptoms and the remainder experiencing unchanged symptoms 180 .
Reinfections are increasingly common 181 . The impact of multiple instances of COVID-19, including the rate of long COVID in those who recovered from a first infection but developed long COVID following reinfection, and the impact of reinfection on those with pre-existing long COVID is crucial to understand to inform future policy decisions. Early research shows an increasing risk of long COVID sequelae after the second and third infection, even in double-vaccinated and triple-vaccinated people 182 . Existing literature suggests multiple infections may cause additional harm or susceptibility to the ME/CFS-type presentation 33 , 183 .
There is also early evidence that certain immune responses in people with long COVID, including low levels of protective antibodies and elevated levels of autoantibodies, may suggest an increased susceptibility to reinfection 27 .
Challenges and recommendations
Issues with PCR and antibody testing throughout the pandemic, inaccurate pandemic narratives and widespread lack of postviral knowledge have caused downstream issues and biases in long COVID research and care.
Testing issues
Most patients with COVID-19 from the first waves did not have laboratory-confirmed infection, with PCR tests being difficult to access unless individuals were hospitalized. Only 1–3% of cases to March 2020 were likely detected 184 , and the CDC estimates that only 25% of cases in the USA were reported from February 2020 to September 2021 (ref. 185 ); that percentage has likely decreased with the rise in use of at-home rapid tests.
Although PCR tests are our best tool for detecting SARS-CoV-2 infections, their false negative rates are still high 186 . Further bias is caused by false negative rates being higher in women and adults younger than 40 years 187 , those with a low viral load 188 and children (Box 1 ), with several studies showing 52–90% of cases in children missed by PCR tests 189 , 190 . The high false negative PCR rate results in symptomatic patients with COVID-19, who seek a COVID-19 test but receive a false negative result, being included as a control in many studies. Those who have a positive PCR test result (who are more likely to be included in research) are more likely to be male or have a higher viral load. Additionally, the lack of test accessibility as well as the false negative rates has created a significant barrier to care, as many long COVID clinics require PCR tests for admission.
Similarly, there is a broad misconception that everyone makes and retains SARS-CoV-2 antibodies, and many clinicians and researchers are unaware of the limited utility of antibody tests to determine prior infection. Between 22% and 36% of people infected with SARS-CoV-2 do not seroconvert, and many others lose their antibodies over the first few months, with both non-seroconversion and seroreversion being more likely in women, children and individuals with mild infections 52 , 53 , 191 , 192 , 193 . At 4 and 8 months after infection, 19% and 61% of patients, respectively, who had mild infections and developed antibodies were found to have seroreverted, compared with 2% and 29% of patients, respectively who had severe infections 191 . Still, many clinicians and researchers use antibody tests to include or exclude patients with COVID-19 from control groups.
Furthermore, during periods of test inaccessibility, tests were given on the basis of patients having COVID-19-specific symptoms such as loss of smell and taste, fever, and respiratory symptoms, resulting in a bias towards people with those symptoms.
Misinformation on PCR and antibody tests has resulted in the categorization of patients with long COVID into non-COVID-19 control groups, biasing the research output. Because low or no antibody levels and viral load may be related to long COVID pathophysiology, including a clinically diagnosed cohort will strengthen the research.
Important miscues
The narrative that COVID-19 had only respiratory sequelae led to a delayed realization of the neurological, cardiovascular and other multisystem impacts of COVID-19. Many long COVID clinics and providers still disproportionately focus on respiratory rehabilitation, which results in skewed electronic health record data. Electronic health record data are also more comprehensive for those who were hospitalized with COVID-19 than for those who were in community care, leading to a bias towards the more traditional severe respiratory presentation and less focus on non-hospitalized patients, who tend to have neurological and/or ME/CFS-type presentations.
The narrative that initially mild COVID-19 cases, generally defined as not requiring hospitalization in the acute phase, would not have long-term consequences has also had downstream effects on research. These so-called mild cases that result in long COVID often have an underlying biology different from acute severe cases, but the same types of tests are being used to evaluate patients. This is despite basic tests such as D-dimer, C-reactive protein (CRP) and antinuclear antibody tests and complete blood count being known to often return normal results in patients with long COVID. Tests that return abnormal results in patients with ME/CFS and dysautonomia, such as total immunoglobulin tests, natural killer cell function tests, the tilt table or NASA lean test, the four-point salivary cortisol test, reactivated herpesvirus panels, small fibre neuropathy biopsy, and tests looking for abnormal brain perfusion 96 , should instead be prioritized. Other recurring issues include studies failing to include the full range of symptoms, particularly neurological and reproductive system symptoms, and not asking patients about symptom frequency, severity and disability. Cardinal symptoms such as postexertional malaise are not widely known, and therefore are rarely included in study designs.
Widespread lack of postviral knowledge and misinformation
The widespread lack of knowledge of viral-onset illnesses, especially ME/CFS and dysautonomia, as well as often imperfect coding, prevents these conditions from being identified and documented by clinicians; this means that they are frequently absent from electronic health record data. Further, because ME/CFS and dysautonomia research is not widely known or comprehensively taught in medical schools 194 , long COVID research is often not built on past findings, and tends to repeat old hypotheses. Additionally, long COVID research studies and medical histories tend to document only the risk factors for severe acute COVID-19, which are different from the risk factors for conditions that overlap with long COVID such as ME/CFS and dysautonomia (for example, connective tissue disorders such as Ehlers–Danlos syndrome, prior illnesses such as infectious mononucleosis and mast cell involvement) 33 , 195 , 196 .
Clinicians who are not familiar with ME/CFS and dysautonomia often misdiagnose mental health disorders in patients; four in five patients with POTS receive a diagnosis with a psychiatric or psychological condition before receiving a POTS diagnosis, with only 37% continuing to have the psychiatric or psychological diagnosis once they have received their POTS diagnosis 117 . Researchers who are unfamiliar with ME/CFS and dysautonomia often do not know to use specific validated tools when conducting mental health testing, as anxiety scales often include autonomic symptoms such as tachycardia, and depression scales often include symptoms such as fatigue, both of which overestimate mental health disorder prevalence in these conditions 197 , 198 .
Recommendations
Although research into long COVID has been expansive and has accelerated, the existing research is not enough to improve outcomes for people with long COVID. To ensure an adequate response to the long COVID crisis, we need research that builds on existing knowledge and is inclusive of the patient experience, training and education for the health-care and research workforce, a public communication campaign, and robust policies and funding to support research and care in long COVID.
We need a comprehensive long COVID research agenda that builds on the existing knowledge from ME/CFS, dysautonomia and other viral-onset conditions, including but not limited to brain and brainstem inflammation, appropriate neuroimaging techniques, neuroimmunology, metabolic profiling, impaired endothelial function, mitochondrial fragmentation, antiviral and metabolic phenotypes, hypoperfusion/cerebral blood flow, nanoneedle diagnostic testing, overlaps with connective tissue disorders, autoimmunity and autoantibodies, viral/microbial persistence, intracranial hypertension, hypermobility, craniocervical obstructions, altered T and B cells, metabolomics and proteomics, elevated blood lactate level, herpesvirus reactivations, immune changes in the early versus late postviral years, and changes to the gut microbiota. The mechanisms of and overlaps between long COVID and connective tissue involvement, mast cells and inflammatory conditions such as endometriosis are particularly understudied and should be focused on. Because of the high prevalence of ME/CFS, POTS and other postinfectious illnesses in patients with long COVID, long COVID research should include people who developed ME/CFS and other postinfectious illnesses from a trigger other than SARS-CoV-2 in comparator groups to improve understanding of the onset and pathophysiology of these illnesses 113 . Additionally, there is a known immune exhaustion process that occurs between the second and third year of illness in ME/CFS, with test results for cytokines being different between patients who have been sick for shorter durations (less than 2 years) than for those who have been sick for longer durations 43 . Because of this, studies should implement subanalyses based on the length of time participants have been ill. Because ME/CFS and dysautonomia research is not widely known across the biomedical field, long COVID research should be led by experts from these areas to build on existing research and create new diagnostic and imaging tools.
Robust clinical trials must be a priority moving forward as patients currently have few treatment options. In the absence of validated treatment options, patients and physicians conduct individual experiments, which result in the duplication of efforts without generalizable knowledge and pose undue risks to patients. Robust study design and knowledge sharing must be prioritized by both funding institutions and clinician-researchers.
It is critical that research on long COVID be representative of (or oversample) the populations who had COVID-19 and are developing long COVID at high rates, which is disproportionately people of colour 35 . Medical research has historically under-represented these populations, and over-representation of white and socio-economically privileged patients has been common in long COVID research. Researchers must work within communities of colour, LGBTQ+ communities and low-income communities to build trust and conduct culturally competent studies that will provide insights and treatments for long COVID for marginalized populations.
As a subset of patients will improve over time, and others will have episodic symptoms, care should be taken to incorporate the possibility of alleviation of symptoms into the study design, and care should be taken not to ascribe improvement to a particular cause without proper modelling.
Finally, it is critical that communities of patients with long COVID and associated conditions are meaningfully engaged in long COVID research and clinical trials. The knowledge of those who experience an illness is crucial in identifying proper study design and key research questions and solutions, improving the speed and direction of research.
Training and education of the health-care and research workforce
To prepare the next generation of health-care providers and researchers, medical schools must improve their education on pandemics, viruses and infection-initiated illnesses such as long COVID and ME/CFS, and competency evaluations should include these illnesses. As of 2013, only 6% of medical schools fully cover ME/CFS across the domains of treatment, research and curricula, which has created obstacles to care, accurate diagnosis, research and treatment 194 . To ensure people with long COVID and associated conditions can receive adequate care now, professional societies and government agencies must educate the health-care and research workforce on these illnesses, including the history of and current best practices for ME/CFS to not repeat mistakes of the past, which have worsened patients’ prognoses. The research community has made a misstep in its efforts to treat ME/CFS 199 , and some physicians, poorly educated in the aetiology and pathophysiology of the disorder, still advise patients to pursue harmful interventions such as graded exercise therapy and cognitive behavioural therapy, despite the injury that these interventions cause 200 and the fact that they are explicitly not advised as treatments 163 , 164 , 166 .
Public communications campaign
In addition to providing education on long COVID to the biomedical community, we need a public communications campaign that informs the public about the risks and outcomes of long COVID.
Policies and funding
Finally, we need policies and funding that will sustain long COVID research and enable people with long COVID to receive adequate care and support. For instance, in the USA, the creation of a national institute for complex chronic conditions within the NIH would go a long way in providing a durable funding mechanism and a robust research agenda. Further, we need to create and fund centres of excellence, which would provide inclusive, historically informed and culturally competent care, as well as conduct research and provide medical education to primary care providers. Additionally, research and clinical care do not exist in silos. It is critical to push forward policies that address both the social determinants of health and the social support that is needed for disabled people.
Conclusions
Long COVID is a multisystemic illness encompassing ME/CFS, dysautonomia, impacts on multiple organ systems, and vascular and clotting abnormalities. It has already debilitated millions of individuals worldwide, and that number is continuing to grow. On the basis of more than 2 years of research on long COVID and decades of research on conditions such as ME/CFS, a significant proportion of individuals with long COVID may have lifelong disabilities if no action is taken. Diagnostic and treatment options are currently insufficient, and many clinical trials are urgently needed to rigorously test treatments that address hypothesized underlying biological mechanisms, including viral persistence, neuroinflammation, excessive blood clotting and autoimmunity.
Acknowledgements
We would like to thank the long COVID and associated conditions patient and research community and the entire team at Patient-Led Research Collaborative. E.J.T. was supported by National Center for Advancing Translational Sciences (NCATS) grant UL1TR002550.
Author information
Authors and affiliations.
Patient-Led Research Collaborative, New York, NY, USA
Hannah E. Davis
Patient-Led Research Collaborative, Oakland, CA, USA
Lisa McCorkell
Scripps Research Translational Institute, Scripps Research, La Jolla, CA, USA
Julia Moore Vogel & Eric J. Topol
You can also search for this author in PubMed Google Scholar
Contributions
The authors contributed equally to all aspects of the article.
Corresponding author
Correspondence to Eric J. Topol .
Ethics declarations
Competing interests.
The authors declare no competing interests.
Peer review
Peer review information.
Nature Reviews Microbiology thanks Akiko Iwasaki, Ziyad Al-Aly and the other, anonymous, reviewer(s) for their contribution to the peer review of this work.
Additional information
Publisher’s note Springer Nature remains neutral with regard to jurisdictional claims in published maps and institutional affiliations.
Rights and permissions
Springer Nature or its licensor (e.g. a society or other partner) holds exclusive rights to this article under a publishing agreement with the author(s) or other rightsholder(s); author self-archiving of the accepted manuscript version of this article is solely governed by the terms of such publishing agreement and applicable law.
Reprints and permissions
About this article
Cite this article.
Davis, H.E., McCorkell, L., Vogel, J.M. et al. Long COVID: major findings, mechanisms and recommendations. Nat Rev Microbiol 21 , 133–146 (2023). https://doi.org/10.1038/s41579-022-00846-2
Download citation
Accepted : 05 December 2022
Published : 13 January 2023
Issue Date : March 2023
DOI : https://doi.org/10.1038/s41579-022-00846-2
Share this article
Anyone you share the following link with will be able to read this content:
Sorry, a shareable link is not currently available for this article.
Provided by the Springer Nature SharedIt content-sharing initiative
This article is cited by
Variations in respiratory and functional symptoms at four months after hospitalisation due to covid-19: a cross-sectional study.
- Monika Fagevik Olsén
- Louise Lannefors
- Hanna C. Persson
BMC Pulmonary Medicine (2024)
Post-recovery health domain scores among outpatients by SARS-CoV-2 testing status during the pre-Delta period
- Jennifer P. King
- Jessie R. Chung
- Edward A. Belongia
BMC Infectious Diseases (2024)
Transforming health care systems towards high-performance organizations: qualitative study based on learning from COVID-19 pandemic in the Basque Country (Spain)
- Ane Fullaondo
- Irati Erreguerena
- Esteban de Manuel Keenoy
BMC Health Services Research (2024)
Psychometric properties of the COVID-19 Yorkshire Rehabilitation Scale: Post-Covid-19 syndrome in Iranian elderly population
- Negar Tamadoni
- Afsaneh Bakhtiari
- Hossein-Ali Nikbakht
Evaluation of disease severity and prediction of severe cases in children hospitalized with influenza A (H1N1) infection during the post-COVID-19 era: a multicenter retrospective study
- Hai-Feng Liu
- Xiao-Zhong Hu
- Hong-Min Fu
BMC Pediatrics (2024)
Quick links
- Explore articles by subject
- Guide to authors
- Editorial policies
Sign up for the Nature Briefing newsletter — what matters in science, free to your inbox daily.


IMAGES
COMMENTS
In simplified terms, the GWAS can be viewed as hypothesis-generating and the CGAS as hypothesis-testing approaches ... COVID-19 Host Genetics Initiative (2020) The COVID-19 host genetics initiative a global initiative to elucidate the role of host genetic factors in susceptibility and severity of the SARS-CoV-2 virus pandemic.
Hypothesis Testing is commonly used when trials for vaccine testing are performed. With all the recent vaccine news for Covid-19, I wanted to shed light on the general hypothesis testing process for vaccines. In this article, I will go over the following topics: · Why is Hypothesis Testing Even Needed for Vaccines?
In late November 2020, there was a flurry of media coverage of two companies' claims of 95% eficacy rates of newly developed COVID-19 vaccines, but information about the confidence interval was not reported. This paper presents a way of teaching the concept of hypothesis testing and the construction of confidence intervals using numbers ...
The enormous U.S. toll of Covid-19 is attributable in part to the lack of an effective testing regimen. ... The more we delay prioritizing and creating the infrastructure needed for hypothesis ...
The COVID lab-leak hypothesis: what scientists do and don't know ... COVID-19 could have come from a lab in a few ways. Researchers might have collected SARS-CoV-2 from an animal and maintained ...
Efficient COVID-19 tests should have low false negative rates to avoid releasing infected people and infecting other people. ... Cohen, K. & Zhao, Q. Active hypothesis testing for anomaly detection.
Population-scale testing is an essential component of responses to the COVID-19 pandemic and is likely to become increasingly important in public health. Here, Mercer and Salit describe the roles ...
Safe, effective vaccines against coronavirus disease 2019 (Covid-19) ... with formal noninferiority hypothesis testing. The immunobridging subset of 5-to-11-year-olds (those whose neutralizing ...
This underscores the pressing need for policymakers to implement COVID-19 testing regimes designed to measure population-level trends in regional infection intensity. Read the full updated PDF her e .
In late November 2020, there was a flurry of media coverage of two companies' claims of 95% efficacy rates of newly developed COVID-19 vaccines, but information about the confidence interval was not reported. This paper presents a way of teaching the concept of hypothesis testing and the construction of confidence intervals using numbers announced by the drug makers Pfizer and Moderna ...
7) Patients will become consumers. The Covid-19 pandemic literally impacts each and every one of us. The boundary between "healthy" and "sick" becomes blurred. People who have otherwise ...
In a dose-escalation study of the COVID-19 RNA vaccine BNT162b1 in 45 healthy adults, RBD-binding IgG concentrations and SARS-CoV-2 neutralizing titres in sera increased with dose level and after ...
Figure.2 A walkthrough using the hypothesis testing (and p-value) to test the null hypothesis that there is no difference in the time of recovery between drug A and placebo. After the test, we reject the null hypothesis. Example 1. Mask wearing and the risk of non-contact transmission in a hamster model for COVID19.
The ongoing COVID-19 pandemic, caused by the novel severe acute respiratory syndrome coronavirus type 2 (SARS-CoV-2), has affected 212 countries worldwide at various degrees as of 8 May 2020.1 In this paper we discuss a hypothesis that prior viral infections—either by SARS-CoV-2 or different strains of coronaviruses, or potentially even other respiratory viruses—may predispose to more ...
In this blog, I'll explain both techniques using recent covid-19 related use-cases and will attempt to drive home the similarities and differences between the two theories. ... Hypothesis Testing.
Testing environmental water to monitor COVID-19 spread in unsheltered encampments. ScienceDaily . Retrieved April 6, 2024 from www.sciencedaily.com / releases / 2024 / 04 / 240403130537.htm
Updated April 4, 2024. Follow the instructions on the Public Health Flowchart for COVID-19 and Respiratory Virus Symptoms if you feel sick, test positive, or are exposed, and know when to report illness.. The University's COVID-19 Health and Safety Plan contains additional requirements and guidance for reducing the risk of COVID-19 transmission in the workplace.
Story by Paul Livengood. • 2d. A n Irving man was sentenced to seven years in prison for his role in a $7 million COVID-19 testing fraud, federal officials announced Thursday. Terrance Barnard ...
Long COVID is an often debilitating illness that occurs in at least 10% of severe acute respiratory syndrome coronavirus 2 (SARS-CoV-2) infections. More than 200 symptoms have been identified with ...
COVID-19 testing is a key strategy that can be utilized by summer camps to identify COVID -19 cases early and prevent transmission from occurring in the camp setting. KDHE strongly recommends . summer camps offer COVID -19 testing for all participants and staff. Free COVID-19 testing resources are available for summer camps through KDHE.